Nervous System Development
Immunology Background
Background
The development of the nervous system is one of the most complex and finely tuned processes in human embryogenesis. It involves the formation of a network of specialized cells that are responsible for controlling the sensory, motor, and cognitive functions of the body. The nervous system can be broadly divided into two major parts: the central nervous system (CNS), which consists of the brain and spinal cord, and the peripheral nervous system (PNS), which includes all other neural elements such as peripheral nerves and ganglia. Together, these systems form the intricate communication network that coordinates bodily functions and responses to external stimuli.
The formation of the nervous system, known as neurogenesis, begins very early in embryonic development and involves a series of highly regulated steps, including neural induction, patterning, cell differentiation, axonal guidance, and synapse formation.
Neural Induction: The Beginning of the Nervous System
The development of the nervous system begins with the process of neural induction, which occurs during gastrulation. At this stage, the embryo consists of three germ layers: ectoderm, mesoderm, and endoderm. The nervous system arises from the ectoderm, the outermost layer of the embryo. However, not all ectodermal cells are destined to become neural tissue; the induction of neural fate is controlled by signaling molecules secreted by the underlying mesodermal tissue, particularly the notochord.
The neural plate forms as ectodermal cells receive signals from the notochord and other adjacent tissues. This process is largely regulated by inhibition of bone morphogenetic protein (BMP) signaling. BMP normally promotes the formation of epidermal tissue, but its inhibition by molecules such as Noggin, Chordin, and Follistatin allows ectodermal cells to adopt a neural fate. The cells of the neural plate eventually give rise to the brain, spinal cord, and other components of the nervous system.
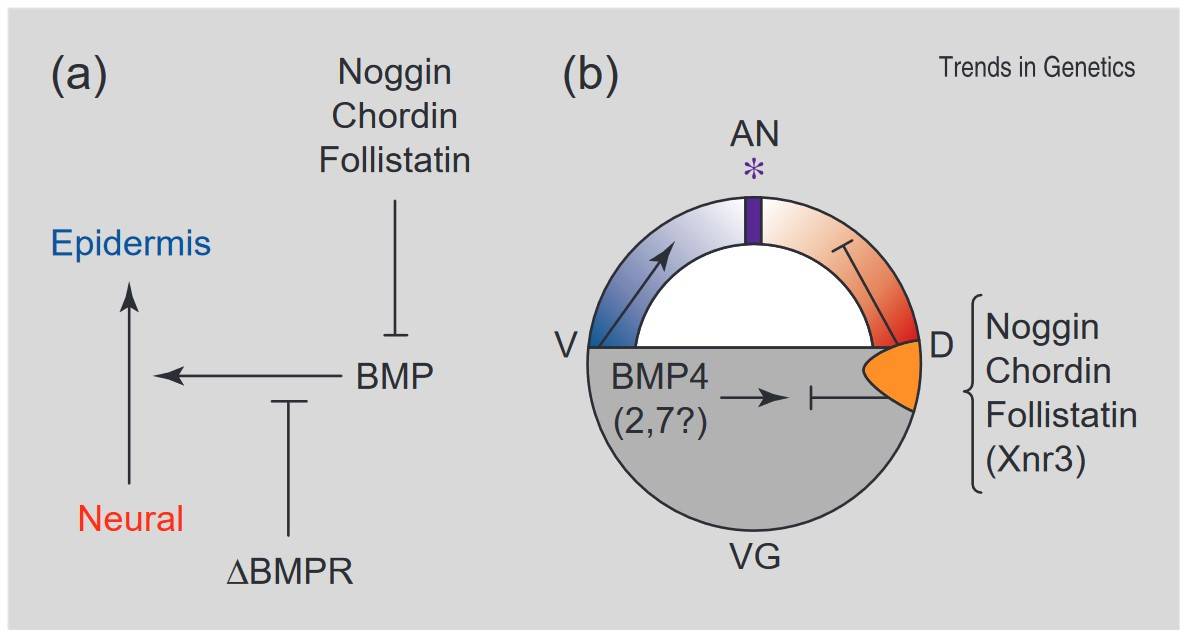
Neurulation: Formation of the Neural Tube
Following neural induction, the neural plate undergoes a process known as neurulation, during which it folds to form the neural tube, the precursor to the CNS. Neurulation occurs in two phases: primary neurulation and secondary neurulation.
- Primary neurulation involves the bending of the neural plate at specific hinge points, which causes the edges of the plate to elevate and fuse, forming the neural tube. This process occurs from the cranial (head) to the caudal (tail) end of the embryo. By the end of primary neurulation, the neural tube is closed, except at the cranial and caudal ends, which will later form the neuropores.
- Secondary neurulation involves the formation of the caudal end of the neural tube through the condensation of mesodermal cells into a solid structure that subsequently cavitates.
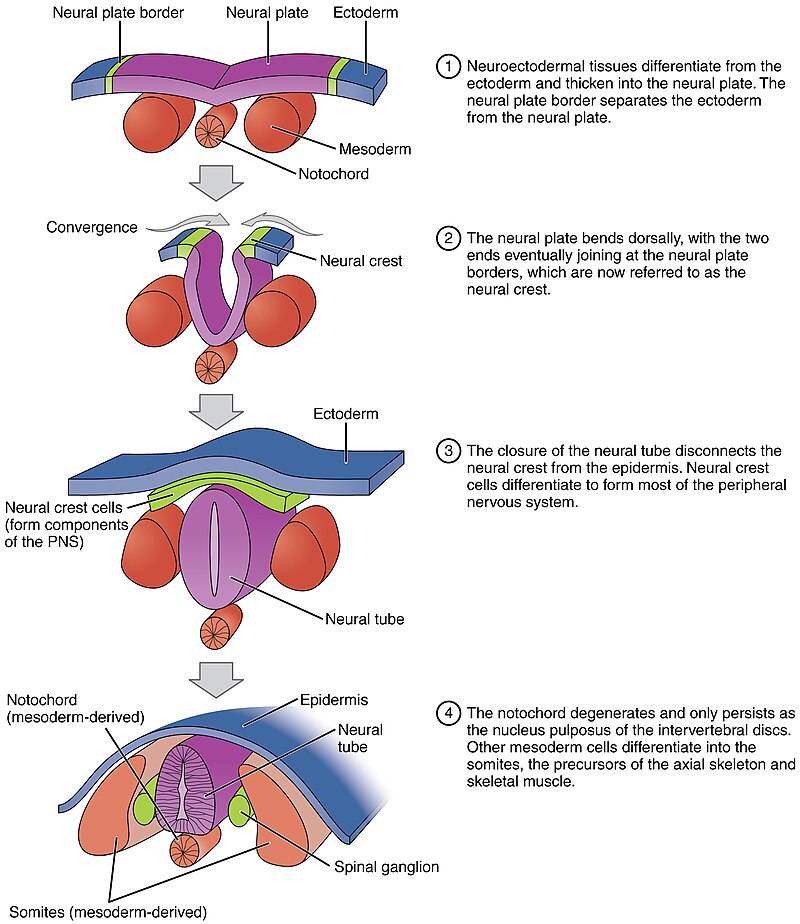
Neurulation is highly dependent on the proper regulation of cell proliferation, migration and adhesion. Disruptions in these processes can lead to neural tube defects (NTDs) such as spina bifida and anencephaly. These defects occur when the neural tube fails to close properly and can have serious consequences for neural development and function.
Regionalization and Patterning of the Neural Tube
Once the neural tube is formed, it undergoes a process of regionalization to give rise to distinct structures in the CNS. This patterning is regulated by gradients of signaling molecules that establish positional information along the anterior-posterior (AP) and dorsal-ventral (DV) axes of the neural tube.
- Anterior-Posterior Patterning: The AP axis determines the division of the neural tube into the forebrain, midbrain, hindbrain, and spinal cord. This regionalization is controlled by signaling molecules such as Wnt and retinoic acid (RA), which establish opposing gradients along the AP axis. The anterior part of the neural tube, which forms the brain, is also influenced by signaling from organizer regions such as the anterior neural ridge (ANR) and the isthmic organizer (IsO). These regions secrete fibroblast growth factors (FGFs) and sonic hedgehog (Shh) to establish distinct brain territories.
- Dorsal-Ventral Patterning: The DV axis determines the organization of different types of neurons within the spinal cord. Sonic Hedgehog (Shh) is secreted by the notochord and the floor plate (ventral part of the neural tube), establishing a ventralizing signal that promotes the formation of motor neurons. In contrast, the roof plate (dorsal part of the neural tube) secretes BMP and Wnt, which promote the formation of sensory neurons in the dorsal region.
These signaling gradients activate specific transcription factors in neural progenitor cells, which then differentiate into distinct types of neurons depending on their position along the AP and DV axes. For example, motor neurons are specified by the expression of the transcription factor Olig2, while sensory neurons express Pax3 and Pax7.
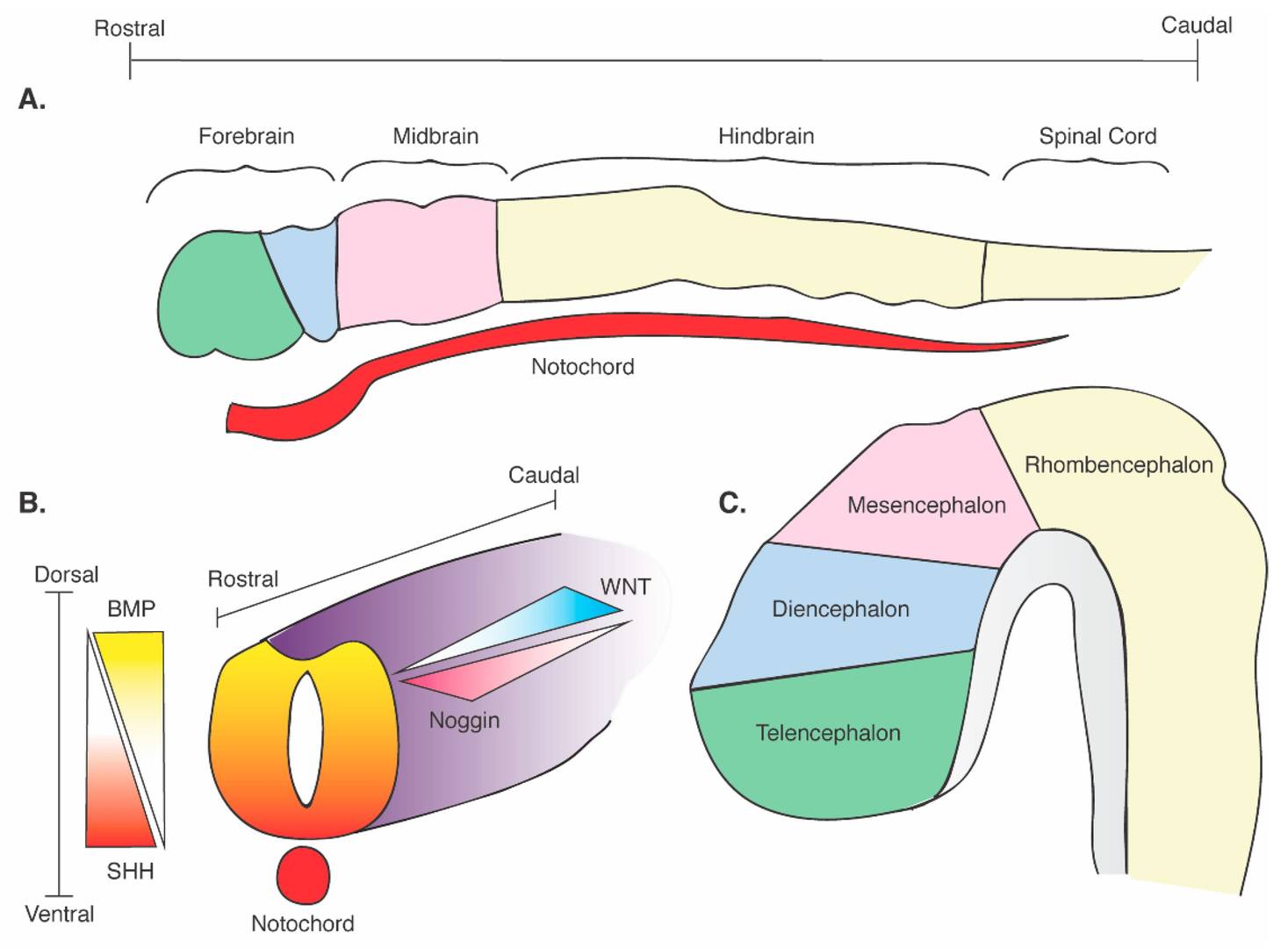
Neural Crest Development
Another key component of nervous system development is the formation of the neural crest, a transient population of multipotent cells that arise at the junction of the neural tube and the epidermis. Neural crest cells undergo an epithelial-to-mesenchymal transition (EMT) that allows them to migrate to different parts of the embryo and differentiate into a variety of cell types, including sensory neurons, autonomic neurons, Schwann cells (glial cells of the PNS), and melanocytes (pigment cells of the skin).
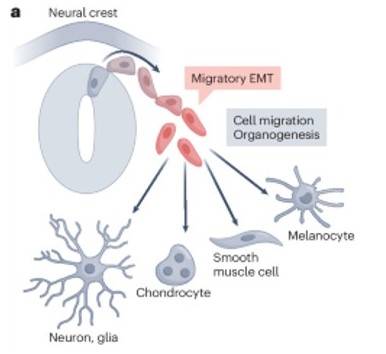
The development of neural crest cells is regulated by a combination of Wnt, BMP and Notch signaling pathways that control their specification, migration and differentiation. Disruptions in neural crest development can lead to a variety of disorders known as neurocristopathies, including Hirschsprung's disease, Waardenburg syndrome, and neurofibromatosis.
Neurogenesis: Generation of Neurons and Glial Cells
Once regionalization and patterning have occurred, the neural progenitor cells within the neural tube begin to proliferate and differentiate into neurons and glial cells, the two main cell types of the nervous system. Neurogenesis, the process of generating neurons, occurs in two main stages: proliferation and differentiation.
- Proliferation: Neural progenitor cells, also known as neuroepithelial cells, initially proliferate rapidly to expand the pool of progenitor cells. These cells are located in the ventricular zone of the neural tube, where they undergo symmetric divisions to produce two identical progenitors.
- Differentiation: After a period of proliferation, neuroepithelial cells begin to divide asymmetrically, giving rise to a progenitor and a differentiated neuron. The newly generated neurons migrate away from the ventricular zone to their final destination in the developing brain or spinal cord. This migration is guided by radial glial cells, which serve as a scaffold for neuronal migration. Once at their destination, neurons extend axons and dendrites to form synaptic connections with other neurons, thereby establishing the neural circuits that underlie brain function.
In addition to neurons, neuroepithelial cells also give rise to glial cells, including astrocytes and oligodendrocytes. Astrocytes provide structural and metabolic support for neurons, while oligodendrocytes are responsible for myelinating axons in the CNS, allowing for rapid transmission of electrical signals.
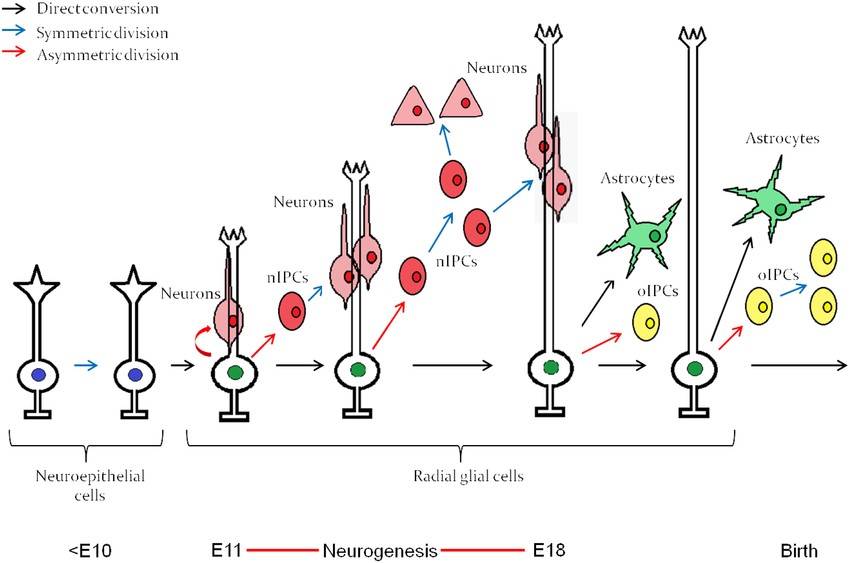
Synaptogenesis and Axonal Guidance
As neurons differentiate and migrate, they begin to form synapses, the specialized connections through which neurons communicate with each other. This process, known as synaptogenesis, involves the precise guidance of axons to their appropriate postsynaptic partners. Axonal guidance is regulated by a variety of molecular cues, including attractive and repulsive signals that guide the growth cone, a specialized structure at the tip of the axon, to its target.
Key molecules involved in axonal guidance include netrins, slits, ephrins, and semaphorins. These molecules bind to receptors on the surface of the growth cone and activate intracellular signaling pathways that regulate cytoskeletal dynamics and direct axon growth. Disruptions in axonal guidance can lead to errors in neural circuit formation, which can result in neurological disorders such as autism and schizophrenia.
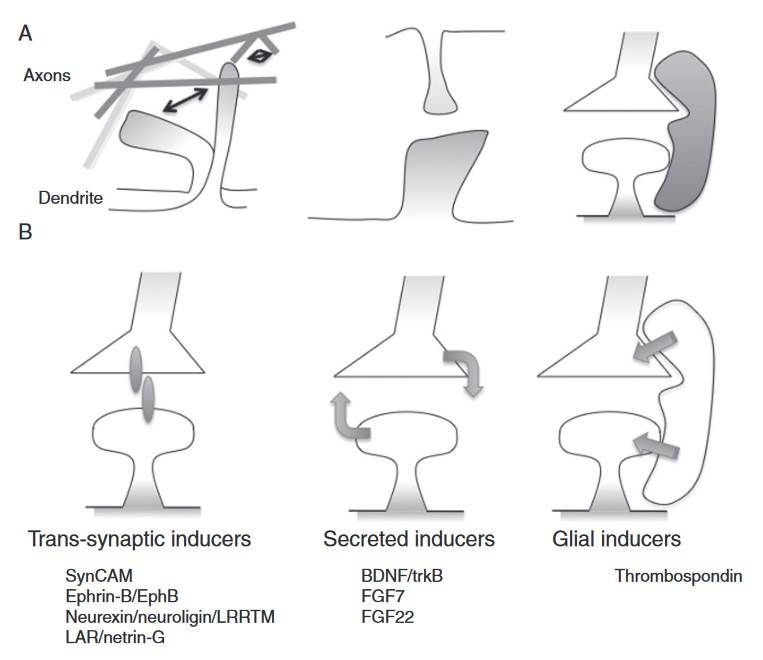
Clinical Implications of Nervous System Development
Given the complexity of nervous system development, it is not surprising that many neurological disorders arise from disruptions in neurodevelopmental processes. Conditions such as autism spectrum disorder (ASD), attention deficit hyperactivity disorder (ADHD), schizophrenia, and cerebral palsy are thought to result, at least in part, from abnormalities in neuronal proliferation, migration, or synaptogenesis.
Understanding the molecular mechanisms that regulate nervous system development has important implications for the diagnosis and treatment of these disorders. For example, identifying genetic mutations that disrupt axonal guidance or synapse formation may provide insight into the underlying causes of autism or intellectual disability. In addition, research on neural stem cells and neurogenesis may offer potential therapeutic strategies for repairing damaged neural tissue in conditions such as spinal cord injury or neurodegenerative diseases.
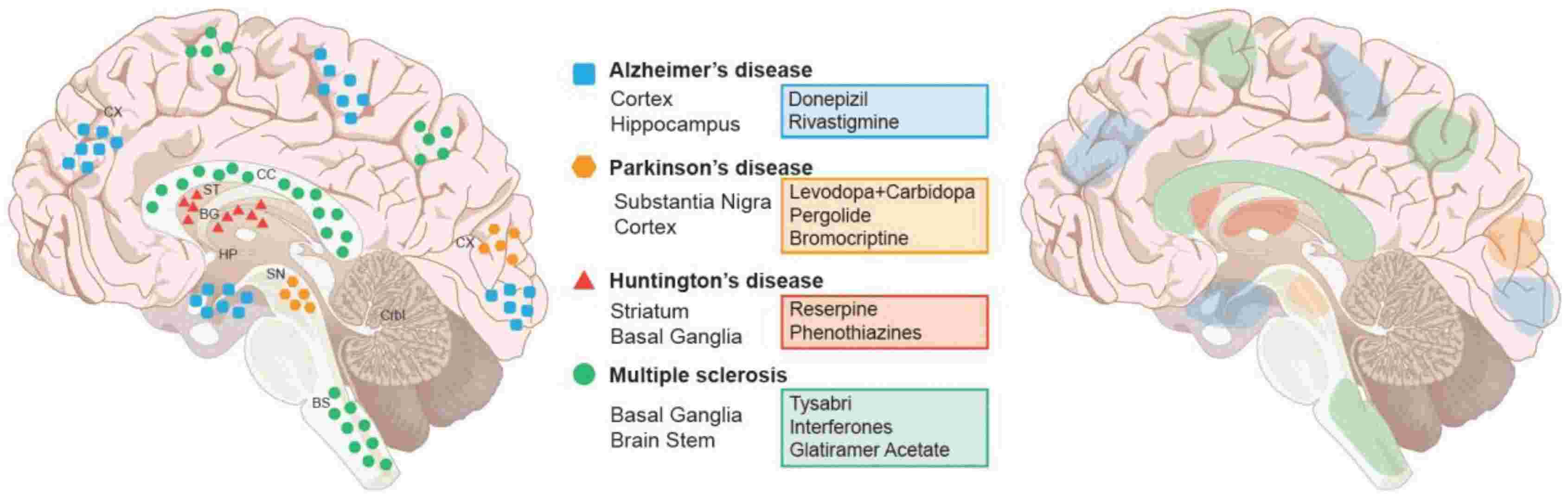
In summary, the development of the nervous system is a highly orchestrated and complex process that begins with neural induction and culminates in the formation of a functional neural network. The intricate interplay of signaling molecules and transcription factors that regulate neurogenesis, patterning, axonal guidance, and synaptogenesis ensures the proper formation and function of the CNS and PNS. Understanding the molecular and cellular mechanisms that underlie nervous system development provides valuable insights into the causes of neurodevelopmental disorders and offers potential avenues for therapeutic intervention.
Case Study
Case 1: Mi, S.; et al. LINGO-1 negatively regulates myelination by oligodendrocytes. Nature Neuroscience. 2005, 8(6), 745–751.
LINGO-1 (Leucine-rich repeat and immunoglobulin-like domain-containing protein 1) has been identified as a key negative regulator of myelination in the central nervous system (CNS), where it is expressed in oligodendrocytes. Suppression of LINGO-1 by dominant-negative LINGO-1, RNA interference, or soluble LINGO-1 (LINGO-1-Fc) enhances oligodendrocyte differentiation and myelination capacity by downregulating RhoA activity, a factor in cell differentiation. Conversely, overexpression of LINGO-1 activates RhoA and inhibits differentiation and myelination. In cocultures of oligodendrocytes and neurons, LINGO-1-Fc treatment promotes robust myelination with developed internodes and nodes of Ranvier. In vivo studies in LINGO-1 knockout mice confirm its regulatory role, suggesting that LINGO-1 signaling is critical for CNS myelination.
The Rho family of GTPases, particularly RhoA, plays a key role in controlling oligodendrocyte differentiation by influencing cellular morphology. Reduced RhoA GTP levels promote oligodendrocyte differentiation. Treatment with LINGO-1-Fc resulted in a significant 70% reduction in RhoA-GTP levels, suggesting that inhibition of LINGO-1 downregulates RhoA, thereby promoting differentiation and increased myelin basic protein (MBP) expression. Similar effects were observed with DN-LINGO-1 and LINGO-1 RNAi treatments. In addition, Fyn kinase, which regulates RhoA, showed increased expression and phosphorylation following DN-LINGO-1 treatment, while FL-LINGO-1 reduced both Fyn expression and phosphorylation by 50%.
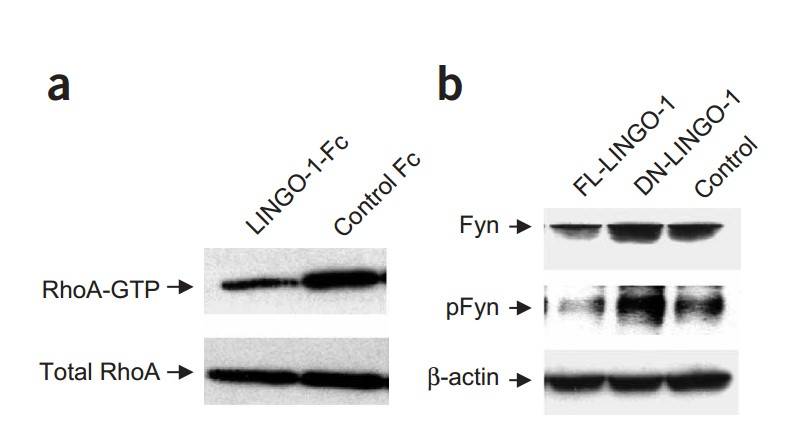
Case 2: Monfrini, E.; et al. Neurofascin (NFASC) gene mutation causes autosomal recessive ataxia with demyelinating neuropathy. Parkinsonism & Related Disorders. 2019, 63, 66–72.
Neurofascin, encoded by NFASC, is a transmembrane protein crucial for nervous system development and node of Ranvier function. Anti-Neurofascin autoantibodies are linked to a specific chronic inflammatory demyelinating polyneuropathy (CIDP) often presenting with cerebellar ataxia and tremor, and recently, homozygous NFASC mutations were linked to a neurodevelopmental disorder in two families. In this study investigating early-onset cerebellar ataxia and demyelinating neuropathy in two siblings from a consanguineous Italian family, researchers used linkage analysis and whole-exome sequencing and identified a homozygous p.V1122E mutation in NFASC. This mutation in a conserved transmembrane domain residue led to a notable reduction of Neurofascin protein in the iPSC-derived neurons of the affected siblings. These findings highlight the role of NFASC mutations in nodopathies, a new class of diseases involving the nodes of Ranvier, and connect these mutations to a hereditary ataxia syndrome with neuropathy for the first time.
Fibroblasts from two affected siblings and three healthy controls were cultured from skin biopsies, reprogrammed into iPSCs, and differentiated into mature neurons. Immunofluorescence after 50 days in vitro showed a distinct neurofascin distribution in control neurons, with concentrated staining in somata and processes. In contrast, patient neurons showed significantly reduced neurofascin immunofluorescence, with less intense, scattered staining in neuronal cell bodies, indicating a substantial loss of neurofascin protein in affected siblings.
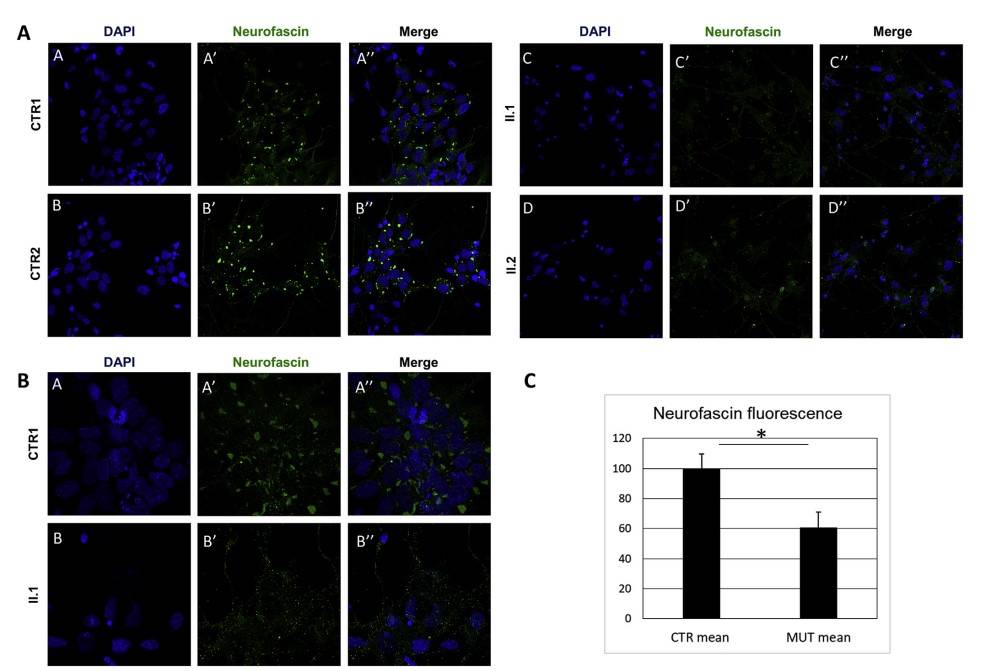
References
- Betts, J. G., Young, K. A., & Wise, J. A. (with Open Textbook Library). (2021). Anatomy and Physiology. 2e, OpenStax, 2021.
- Brady, M. V., & Vaccarino, F. M. (2021). Role of shh in patterning human pluripotent cells towards ventral forebrain fates. Cells, 10(4), 914.
- Hussain, R., Zubair, H., Pursell, S., & Shahab, M. (2018). Neurodegenerative diseases: Regenerative mechanisms and novel therapeutic approaches. Brain Sciences, 8(9), 177.
- Kayser, M. S., & Dalva, M. B. (2014). Synaptogenesis. In G. Kwakkel, L. Cohen, M. Selzer, R. Miller, & S. Clarke (Eds.), Textbook of Neural Repair and Rehabilitation: Volume 1: Neural Repair and Plasticity (2nd ed., Vol. 1, pp. 317–328). Cambridge University Press.
- Mi, S., Miller, R. H., Lee, X., Scott, M. L., Shulag-Morskaya, S., Shao, Z., Chang, J., Thill, G., Levesque, M., Zhang, M., Hession, C., Sah, D., Trapp, B., He, Z., Jung, V., McCoy, J. M., & Pepinsky, R. B. (2005). LINGO-1 negatively regulates myelination by oligodendrocytes. Nature Neuroscience, 8(6), 745–751.
- Mitrousis, N., Tropepe, V., & Hermanson, O. (2015). Post-translational modifications of histones in vertebrate neurogenesis. Frontiers in Neuroscience, 9.
- Monfrini, E., Straniero, L., Bonato, S., Monzio Compagnoni, G., Bordoni, A., Dilena, R., Rinchetti, P., Silipigni, R., Ronchi, D., Corti, S., Comi, G. P., Bresolin, N., Duga, S., & Di Fonzo, A. (2019). Neurofascin (NFASC) gene mutation causes autosomal recessive ataxia with demyelinating neuropathy. Parkinsonism & Related Disorders, 63, 66–72.
- Streit, A., & Stern, C. D. (1999). Neural induction: A bird's eye view. Trends in Genetics, 15(1), 20–24.
- Youssef, K. K., & Nieto, M. A. (2024). Epithelial–mesenchymal transition in tissue repair and degeneration. Nature Reviews Molecular Cell Biology, 25(9), 720–739.