Morphogens, Embryonic Patterning and Axis Formation
Creative BioMart Morphogens, Embryonic Patterning and Axis Formation Product List
Immunology Background
Background
The intricate process of embryonic development relies on a precise set of signals to guide the differentiation of cells into different tissues and organs. A key component of this developmental process is the concept of morphogens - substances that form gradients and provide positional information to cells in developing tissues. These molecules play a critical role in embryonic patterning, which refers to the spatial organization of cells during development. Patterning is closely linked to the formation of body axes, including the anterior-posterior, dorsal-ventral and left-right axes, which determine the overall structure of the organism.
Morphogens: Definition and Mechanism of Action
Morphogens are signaling molecules that regulate the fate of cells based on their concentration within a gradient. These molecules are distributed asymmetrically in developing tissues, and cells exposed to different concentrations of a morphogen respond by activating different genetic programs. The result is the differentiation of cells into different types based on their position relative to the source of the morphogen.
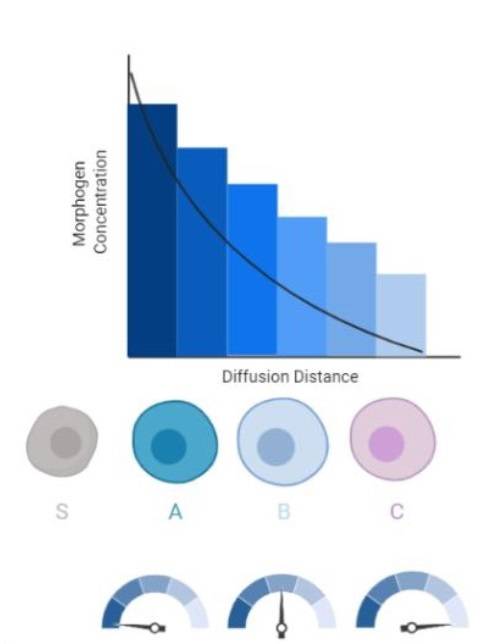
Morphogens operate by binding to receptors on the surfaces of cells, initiating intracellular signaling pathways that ultimately influence gene expression. One of the most well-known morphogen systems is the Sonic Hedgehog (Shh) pathway, which is involved in patterning the neural tube, limbs, and other tissues. Other important morphogens include Bone Morphogenetic Proteins (BMPs), Wnt proteins, and Retinoic Acid (RA), all of which play significant roles in various aspects of embryonic development.
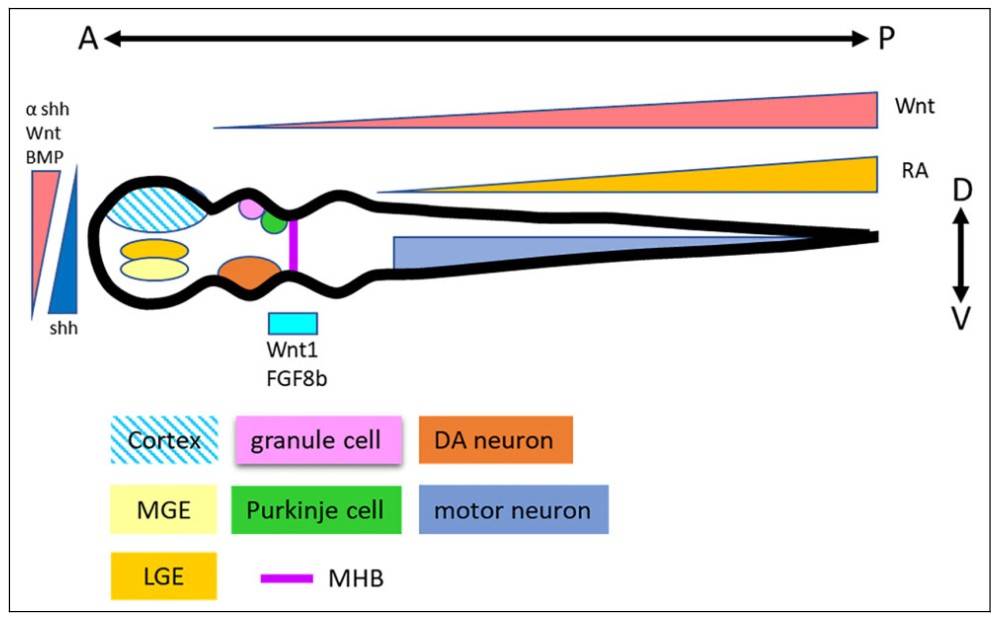
One of the defining features of morphogen action is the ability of cells to interpret different concentration thresholds that lead to different developmental outcomes. This concentration-dependent response is critical for the formation of complex patterns within tissues. For example, in the developing vertebrate neural tube, high concentrations of Shh induce the formation of ventral cell types, whereas lower concentrations induce the differentiation of dorsal cell types.
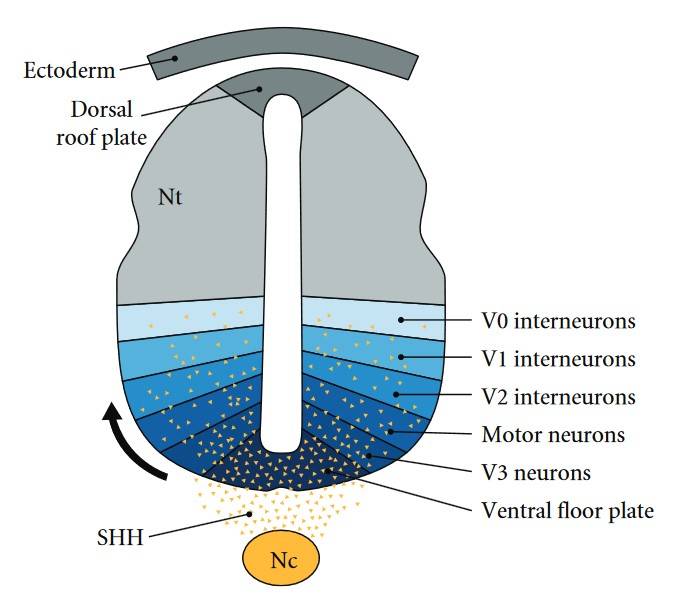
Embryonic Patterning and Morphogen Gradients
Embryonic patterning refers to the process by which cells are organized into distinct regions with specific identities during development. Morphogen gradients are one of the primary mechanisms by which patterning occurs. These gradients provide cells with information about their position within the embryo, allowing them to adopt specific fates that contribute to the overall structure of the organism.
One of the classic examples of morphogen-mediated patterning is found in Drosophila melanogaster (fruit fly) embryogenesis. During early stages of development, a gradient of Bicoid protein establishes the anterior-posterior axis of the embryo. Bicoid is expressed at the anterior end of the embryo and diffuses posteriorly, forming a gradient. Cells in different regions of the embryo interpret this gradient by activating specific genes, such as hunchback, that contribute to the formation of head and thoracic structures. This illustrates how a single morphogen can organize the entire anterior-posterior axis of the embryo.
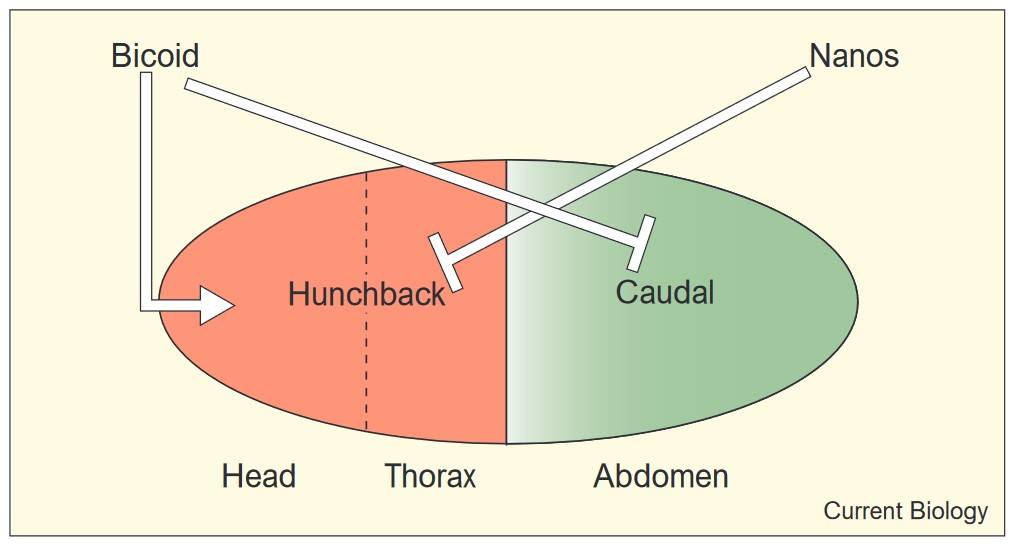
Similar principles apply in vertebrates. The anterior-posterior axis is established by the interaction of multiple signaling pathways, including Wnt, BMP, and FGF (fibroblast growth factor) signaling. Gradients of these morphogens direct the formation of different regions of the neural tube, somites (precursors of vertebrae), and other structures along the length of the body.
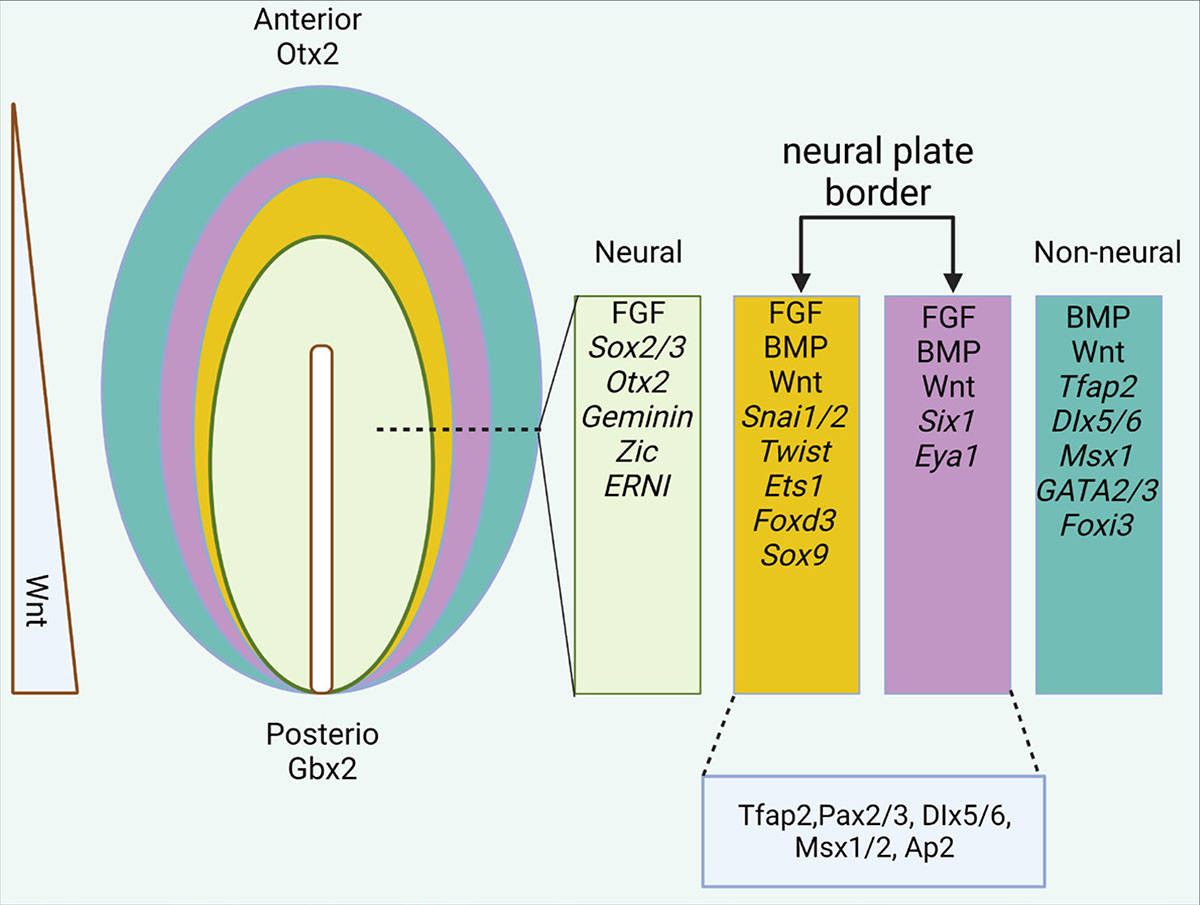
Axis Formation: Anterior-Posterior, Dorsal-Ventral, and Left-Right
The establishment of body axes is one of the earliest and most critical steps in embryonic development. The three primary axes-anterior-posterior (head to tail), dorsal-ventral (back to abdomen), and left-right-are established by the coordinated action of morphogens and signaling pathways.
Anterior-Posterior Axis Formation
The anterior-posterior axis is the first to be established in most organisms. In Drosophila, the Bicoid gradient discussed earlier plays a critical role in anterior-posterior patterning. In vertebrates, the formation of this axis begins with the establishment of the primitive streak, a structure that forms on the surface of the developing embryo and serves as a source of signals that pattern the body.
The Wnt signaling pathway is particularly important in anterior-posterior axis formation in vertebrates. High levels of Wnt signaling at the posterior end of the embryo induce the formation of tail structures, while lower levels at the anterior end contribute to head development. Additionally, retinoic acid plays a role in anterior-posterior patterning by regulating the expression of genes such as Hox genes, which provide cells with positional information along the axis.
Left-Right Axis Formation
The left-right axis is the last to be established and is essential for the asymmetric development of organs such as the heart, lungs, and liver. In vertebrates, left-right axis formation is influenced by the action of cilia, small hair-like structures that generate a flow of signaling molecules on the surface of the embryo. This flow helps to establish asymmetry by generating differential signaling on the left and right sides of the embryo.
One of the key signaling pathways involved in the formation of the left-right axis is the Nodal signaling pathway. Nodal is expressed asymmetrically, with higher levels on the left side of the embryo. This asymmetry is necessary for proper organ positioning. Mutations in genes that disrupt left-right axis formation can lead to conditions such as situs inversus, in which internal organs are mirrored from their normal positions.
Dorsal-Ventral Axis Formation
The dorsal-ventral axis is established perpendicular to the anterior-posterior axis. In Drosophila, the Dorsal protein plays a key role in this process. Dorsal is distributed in a gradient, with higher concentrations on the ventral side of the embryo. Cells exposed to different levels of Dorsal adopt either a dorsal or ventral fate, leading to the differentiation of tissues such as the nervous system on the ventral side and the epidermis on the dorsal side.
In vertebrates, the BMP signaling pathway is critical for the establishment of the dorsal-ventral axis. BMPs are expressed on the ventral side of the embryo, while Noggin and Chordin, which inhibit BMP signaling, are expressed on the dorsal side. This interaction establishes a gradient of BMP activity that patterns the dorsal-ventral axis.
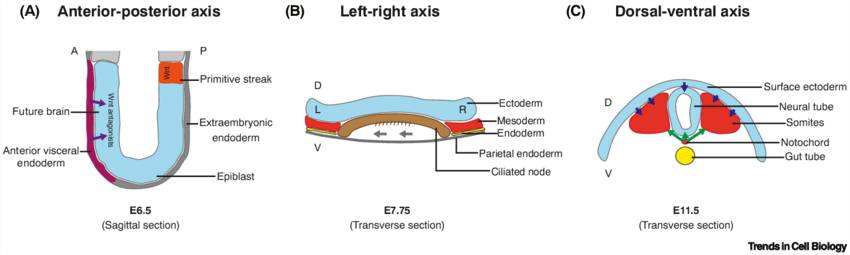
Cross-talk Between Signaling Pathways
While morphogens and their respective pathways are often discussed independently, embryonic development relies on extensive cross-talk between these signaling pathways. For example, in the developing limb bud, the Sonic Hedgehog and FGF pathways interact to regulate the patterning of bones and digits. Similarly, the BMP, Wnt, and Nodal pathways coordinate to ensure proper formation of body axes and tissues.
This cross-talk ensures that cells receive consistent and integrated signals about their position and fate during development. Disruptions in these interactions can lead to developmental defects and congenital disorders.
Applications and Clinical Relevance
The study of morphogens and embryonic patterning has important clinical implications. Many congenital disorders result from disruptions in these pathways. For example, mutations in the Sonic Hedgehog pathway can lead to holoprosencephaly, a condition in which the brain fails to divide properly into two hemispheres. Similarly, disruptions in BMP signaling can cause skeletal malformations and other developmental abnormalities.
In regenerative medicine, understanding how morphogens control cell fate has potential applications in tissue engineering and stem cell therapy. By manipulating morphogen gradients, scientists can direct the differentiation of stem cells into specific cell types, offering the potential to regenerate damaged tissues or organs.
In summary, morphogens, embryonic patterning, and axis formation are central to the development of complex organisms. Through the action of morphogen gradients, cells receive positional information that guides their differentiation into specific tissues and organs. The formation of the anterior-posterior, dorsal-ventral, and left-right axes is tightly regulated by signaling pathways such as Sonic Hedgehog, BMP, Wnt, and Nodal, which interact to ensure the proper organization of the body.
By studying these processes, we gain insight into the fundamental mechanisms of development and their relevance to human health and disease. Understanding how morphogens regulate embryonic patterning not only advances our knowledge of biology but also opens new avenues for medical applications, including the treatment of congenital disorders and the development of regenerative therapies.
Case Study
Case 1: Jablonska, B.; et al. Chordin-induced lineage plasticity of adult SVZ neuroblasts after demyelination. Nature Neuroscience. 2010, 13(5), 541–550.
This study investigates adult neural precursors in the subventricular zone (SVZ) and their developmental plasticity under normal and pathological conditions. In healthy mice, GAD65-positive and Dcx-positive progenitors express Pax6 and migrate to the olfactory bulb to form interneurons. However, after lysolecithin-induced demyelination in the corpus callosum, these progenitors switch from a neuronal to a glial fate and produce oligodendrocytes instead. This switch, which is influenced by upregulated chordin in the SVZ, suggests a potential therapeutic approach for brain injury or disease by exploiting the lineage flexibility of SVZ progenitors.
To determine whether GAD65-GFP-positive cells of the adult SVZ could generate glia rather than neurons under pathological conditions, the researchers analyzed these cells after lysolecithin (LPC)-induced demyelination of the corpus callosum. The results show that while the total number of GAD65-GFP-positive cells remains unchanged after injury, there is an increase in markers associated with oligodendroglial precursors (Mash1, Olig2, and NG2) in these cells. However, markers of neuronal lineage (Dcx, Pax6, and Dlx2) do not show significant changes, suggesting that demyelination enhances the glial potential of GAD65-GFP-positive cells without affecting their neuronal lineage commitment.
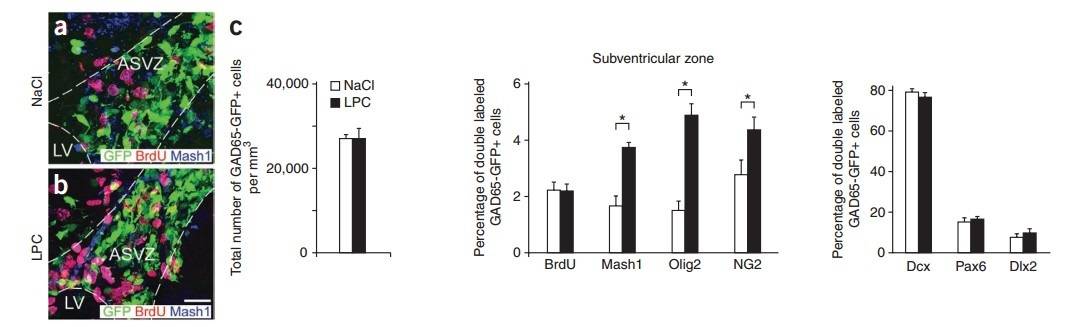
Case 2: Cortés, C. R.; et al. Mutations in human C2CD3 cause skeletal dysplasia and provide new insights into phenotypic and cellular consequences of altered C2CD3 function. Scientific Reports. 2016, 6(1), 24083.
Ciliopathies, caused by defects in the primary cilium, encompass genetic disorders like orofaciodigital syndromes (OFDS) and short rib polydactyly syndromes. Recent research links mutations in C2CD3, a centriolar protein gene, to a new OFDS subtype (OFD14), associated with microcephaly and cerebral issues. In a third family, novel C2CD3 mutations led to skeletal dysplasia without microcephaly in two fetuses. Fibroblast studies from one fetus showed reduced cilia formation, pointing to C2CD3's role in basal body maturation, with CEP164 recruitment alone proving insufficient for full maturation and axonemal extension in C2CD3-deficient cells.
Using fibroblasts from an individual with C2CD3 mutation (G3P1), researchers observed a significant decrease in ciliated cells after serum starvation-induced ciliogenesis, as compared to control juvenile fibroblasts. This was confirmed through ARL13b staining of the axoneme. A fetal fibroblast line from a non-ciliopathy case showed ciliogenesis levels comparable to the juvenile fibroblast control, suggesting age-related differences do not affect ciliogenesis in fibroblasts. These results align with findings in other species, reinforcing C2CD3's role in human ciliogenesis.
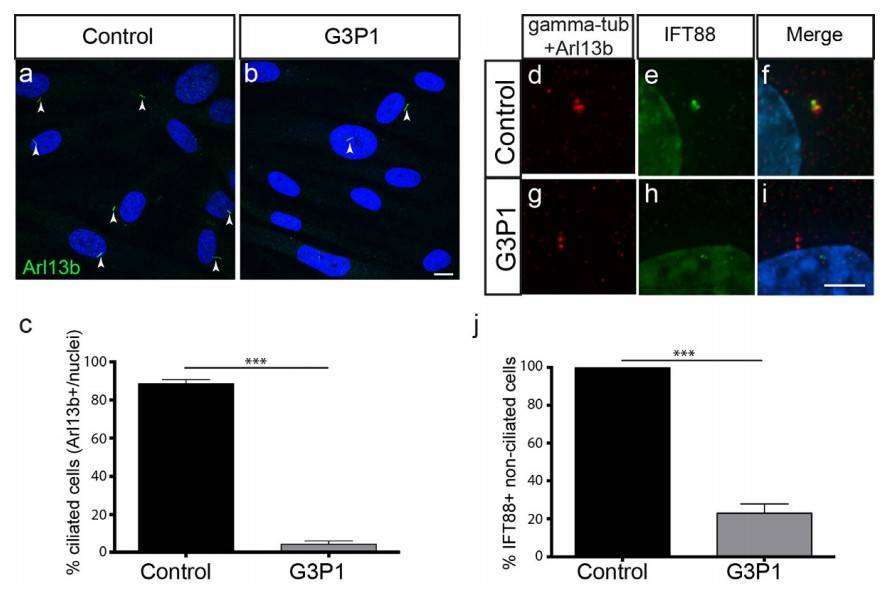
References
- Altyar, A. E., El-Sayed, A., Abdeen, A., Piscopo, M., Mousa, S. A., Najda, A., & Abdel-Daim, M. M. (2023). Future regenerative medicine developments and their therapeutic applications. Biomedicine & Pharmacotherapy, 158, 114131.
- Chang, C.-Y., Ting, H.-C., Liu, C.-A., Su, H.-L., Chiou, T.-W., Harn, H.-J., Lin, S.-Z., & Ho, T.-J. (2021). Differentiation of human pluripotent stem cells into specific neural lineages. Cell Transplantation, 30, 09636897211017829.
- Cortés, C. R., McInerney-Leo, A. M., Vogel, I., Rondón Galeano, M. C., Leo, P. J., Harris, J. E., Anderson, L. K., Keith, P. A., Brown, M. A., Ramsing, M., Duncan, E. L., Zankl, A., & Wicking, C. (2016). Mutations in human C2CD3 cause skeletal dysplasia and provide new insights into phenotypic and cellular consequences of altered C2CD3 function. Scientific Reports, 6(1), 24083.
- Dearden, P., & Akam, M. (1999). Developmental evolution: Axial patterning in insects. Current Biology, 9(16), R591–R594.
- Esmaeli, M., Barazesh, M., Karimi, Z., Roshankhah, S., & Ghanbari, A. (2024). Molecular signaling directing neural plate border formation. The International Journal of Developmental Biology, 68(2), 65–78.
- Gurdon, J. B., & Bourillot, P.-Y. (2001). Morphogen gradient interpretation. Nature, 413(6858), 797–803.
- Jablonska, B., Aguirre, A., Raymond, M., Szabo, G., Kitabatake, Y., Sailor, K. A., Ming, G.-L., Song, H., & Gallo, V. (2010). Chordin-induced lineage plasticity of adult SVZ neuroblasts after demyelination. Nature Neuroscience, 13(5), 541–550.
- Lee, R. W. Y., & Tierney, E. (2011). Hypothesis: The role of sterols in autism spectrum disorder. Autism Research and Treatment, 2011, 1–7.
- Lieblich, S. (2020). "Evolution" of embryogenesis: Complexity of the early developmental stages in the animal kingdom. OBM Genetics, 4(3), 1–29.
- Van Den Brink, S. C., & Van Oudenaarden, A. (2021). 3D gastruloids: A novel frontier in stem cell-based in vitro modeling of mammalian gastrulation. Trends in Cell Biology, 31(9), 747–759.