Histone Deacetylases (HDACs) and Associated Proteins
Related Symbol Search List
Immunology Background
Background
Histone deacetylases (HDACs) are enzymes that catalyze the removal of acetyl groups from lysine residues on histone proteins. They are part of a larger class of enzymes known as histone modifiers, which include histone acetyltransferases (HATs) that add acetyl groups. HDACs counteract the effects of HATs by deacetylating histones, leading to a more condensed chromatin structure and often resulting in gene repression.
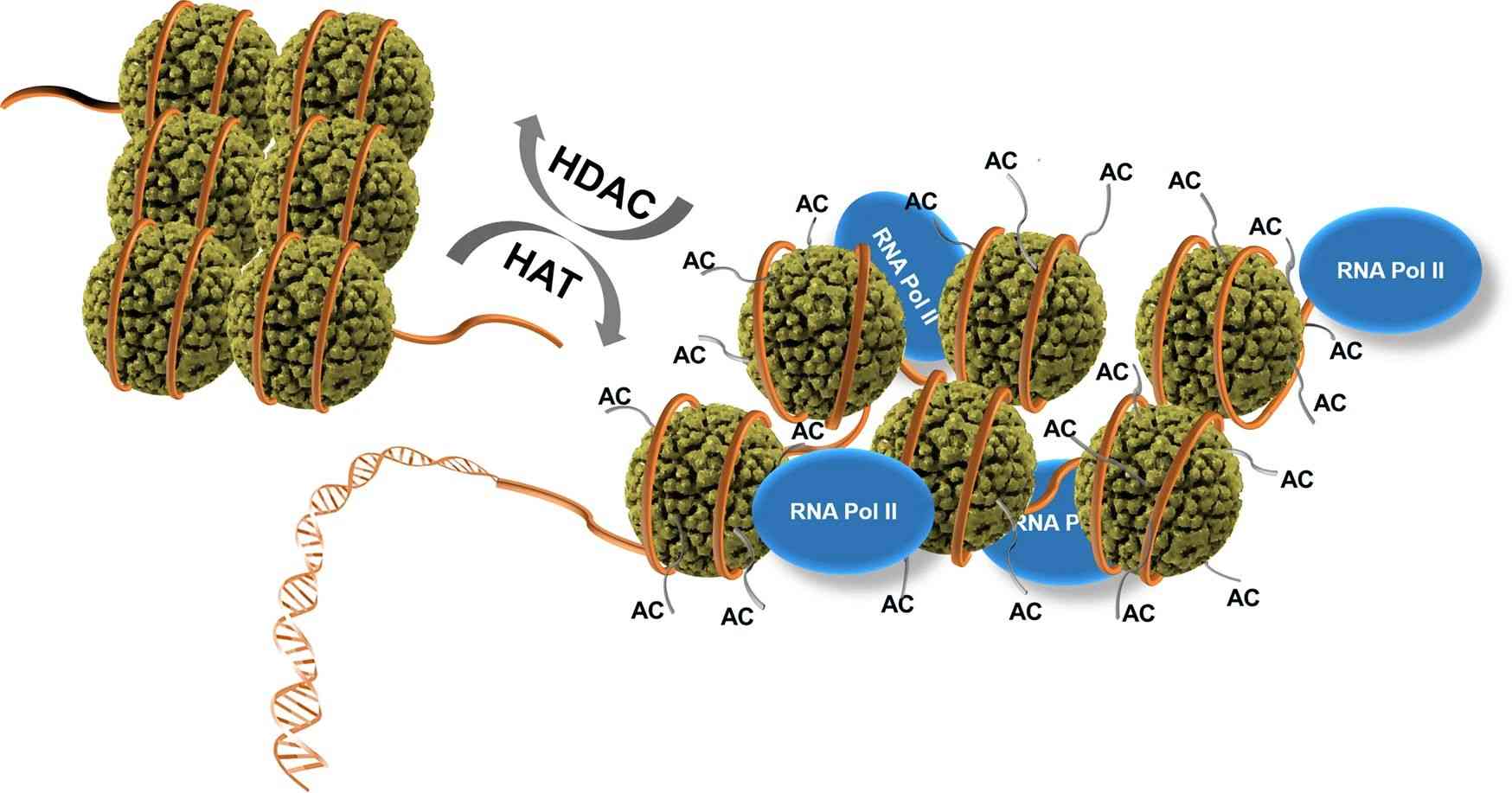
Acetylation (AC) of histone lysine residues by HAT, opening up the chromatin structure, allows binding of RNA polymerase II (RNA Pol II), while deacetylation of the histone lysine residues by HDAC leads to the closed chromatin conformation to be unable to bind RNA Pol II. Histones are displayed with dark green spheres. DNA wound around the histones is shown as an orange tube. The histone lysine residues are drawn with thin and short gray tails on histone spheres.
Here's their role in gene expression regulation, and their importance in various cellular processes:
Role and importance | Details | |
---|---|---|
Role in Gene Expression Regulation | By modifying the acetylation status of histones, HDACs influence the accessibility of DNA to transcriptional machinery. Deacetylation of histones by HDACs promotes a more compact chromatin conformation, making the DNA less accessible for transcription factors and other regulatory proteins. This generally leads to gene repression or silencing. HDACs can also regulate gene expression by interacting with non-histone proteins and modifying their acetylation status. | |
Importance in Various Cellular Processes | Development and differentiation | HDACs play critical roles in embryonic development, tissue-specific differentiation, and cellular maturation. They help regulate the expression of genes involved in development and differentiation processes, such as cell cycle control, stem cell maintenance, and tissue-specific gene expression. |
Cell cycle regulation | HDACs are involved in the control of cell cycle progression by regulating the expression of genes that govern cell cycle checkpoints, DNA repair, and cell cycle inhibitors. Dysregulation of HDAC activity can lead to aberrant cell cycle progression and contribute to diseases such as cancer. | |
Epigenetic regulation | HDACs are key players in the epigenetic control of gene expression. They participate in the establishment and maintenance of epigenetic marks, including DNA methylation and histone modifications, which contribute to stable gene silencing or activation. | |
Chromatin remodeling | HDACs contribute to the remodeling of chromatin structure, influencing DNA accessibility and the formation of higher-order chromatin structures. They interact with other chromatin-modifying enzymes and complexes to regulate the overall chromatin landscape. |
In summary, HDACs are enzymes that remove acetyl groups from histones, contributing to the regulation of gene expression and the modulation of chromatin structure. Their importance extends beyond gene expression control, influencing a wide range of cellular processes, development, and disease pathways. Understanding the functions and dysregulation of HDACs provides insights into the complex mechanisms of gene regulation and opens avenues for therapeutic interventions.
Classification of HDACs
HDACs are classified into four main classes based on their sequence homology, enzymatic activity, and cellular localization. Each class of HDACs has distinct functions and cellular localizations. Here's an overview of the different classes of HDACs and their respective subtypes:
Classification | Details |
---|---|
Class I HDACs | Class I HDACs are primarily localized in the nucleus and consist of four subtypes: HDAC1, HDAC2, HDAC3, and HDAC8. They are ubiquitously expressed and play important roles in various cellular processes, including transcriptional regulation, cell cycle control, and differentiation. Class I HDACs are often found in large multiprotein complexes such as Sin3 and NuRD, where they contribute to gene repression by deacetylating histones. They are involved in the modulation of chromatin structure and the regulation of specific target genes. |
Class II HDACs |
Class II HDACs are divided into two subclasses: Class IIa and Class IIb. They exhibit both nuclear and cytoplasmic localization and have tissue-specific expression patterns. Class IIa HDACs include HDAC4, HDAC5, HDAC7, and HDAC9, while Class IIb HDACs consist of HDAC6 and HDAC10. Class IIa HDACs play important roles in development, cellular differentiation, and tissue-specific gene regulation. They can shuttle between the nucleus and cytoplasm and interact with various transcription factors and co-regulators. Class IIa HDACs are involved in signal-dependent gene expression and are regulated through phosphorylation-mediated nucleocytoplasmic shuttling. HDAC6, a member of Class IIb, is predominantly localized in the cytoplasm and is involved in the regulation of protein quality control, aggresome formation, and microtubule dynamics. HDAC6 can deacetylate non-histone proteins, such as α-tubulin, Hsp90, and cortactin, thereby affecting cellular processes like cell migration, protein degradation, and cell survival. |
Class III HDACs (Sirtuins) | Class III HDACs are also referred to as sirtuins and are distinct from the other classes of HDACs in terms of their enzymatic mechanism and dependency on NAD+ as a cofactor. There are seven mammalian sirtuins: SIRT1-7. Sirtuins are found in various cellular compartments, including the nucleus, cytoplasm, and mitochondria. They are involved in diverse processes, including metabolism, stress response, DNA repair, and aging. Sirtuins regulate gene expression and protein function through deacetylation of both histone and non-histone proteins, including transcription factors and metabolic enzymes. |
Class IV HDA | Class IV HDAC consists of a single member, HDAC11. HDAC11 shares sequence similarity with both Class I and Class II HDACs. It is primarily localized in the nucleus and has been implicated in immune regulation. HDAC11 regulates gene expression through the deacetylation of histones and other protein targets. |
It's important to note that the classification of HDACs is primarily based on sequence homology and enzymatic properties. However, HDACs can exhibit functional redundancy and complex interactions with other proteins and pathways, making their precise roles in cellular processes context-dependent.
Structure of HDACs
HDACs have a conserved catalytic domain known as the HDAC domain, which is responsible for their deacetylase activity. The catalytic domain typically contains a zinc ion coordinated by conserved histidine residues, which is crucial for enzymatic activity. In addition to the catalytic domain, HDACs may also have other domains that contribute to their function and interaction with other proteins. For example, Class I HDACs have an N-terminal extension that contains a nuclear localization signal (NLS) for their nuclear import.
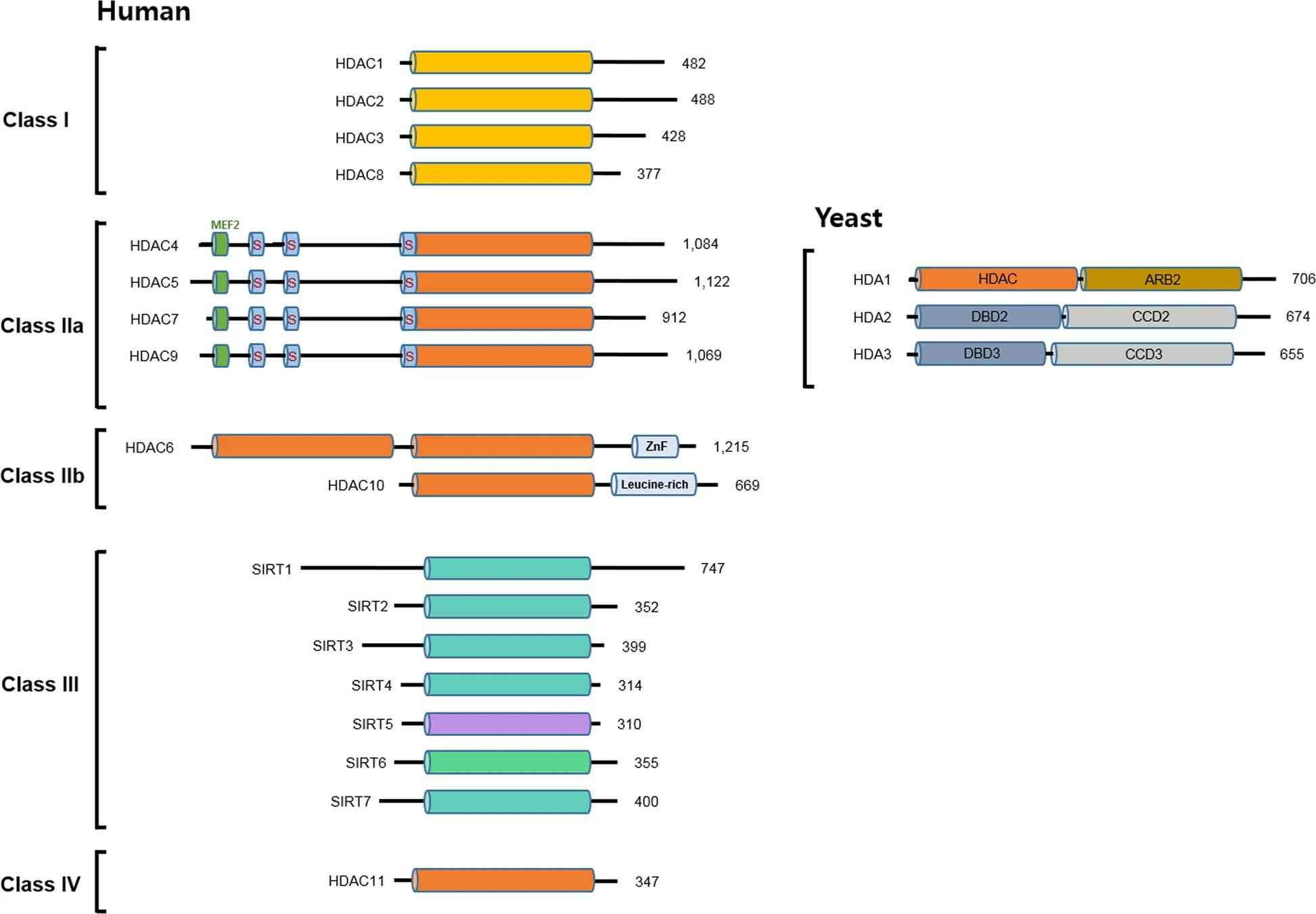
Catalytic mechanism of histone deacetylation
HDACs catalyze the deacetylation of histones by a two-step mechanism. First, they bind to the acetylated lysine residues on histones through their catalytic domain. Then, the zinc ion in the active site of HDACs facilitates the nucleophilic attack of a water molecule on the acetyl group, leading to its removal and the restoration of a positively charged lysine residue. This deacetylation process contributes to the compaction of chromatin and gene repression.
Formation of HDAC complexes
HDACs often associate with other proteins to form large multiprotein complexes, which play important roles in their recruitment to specific genomic loci and regulation of their enzymatic activity. These complexes can include co-repressors, transcription factors, chromatin remodeling factors, and other epigenetic regulators. The formation of complexes allows HDACs to interact with specific DNA sequences or target genes and modulate their expression. For example, Class I HDACs (HDAC1, HDAC2, HDAC3, and HDAC8) are found in large complexes such as the Sin3 and NuRD complexes. Class II HDACs (HDAC4, HDAC5, HDAC6, HDAC7, HDAC9, and HDAC10) can shuttle between the nucleus and cytoplasm and interact with other proteins, including transcription factors and chaperones. Class III HDACs, or sirtuins (SIRT1-7), are NAD+-dependent enzymes that exhibit distinct subcellular localization and can form complexes with other proteins involved in metabolism, stress response, and aging. The association of HDACs with these complexes provides additional layers of regulation and specificity in their targeting to specific genomic regions and coordination with other regulatory factors.
Representative Members of HDACs and Associated Proteins
Members | Classification and function |
---|---|
HDAC1, HDAC2, HDAC3, HDAC8 |
Class: Class I HDAC Function: They are involved in gene regulation, chromatin remodeling, and transcriptional repression.
|
HDAC10 |
Class: Class IIb HDAC Function: HDAC10 is primarily localized in the cytoplasm and is involved in the regulation of protein quality control and cellular processes such as cell migration and protein degradation. HDAC10 can deacetylate non-histone proteins, impacting their function and cellular activities. |
HDAC11 | Class: Class IV HDAC
Function: HDAC11 is primarily localized in the nucleus and has been implicated in immune regulation and inflammatory responses. It plays a role in gene expression regulation by deacetylating histones and other protein targets. |
SIRT1, SIRT2, SIRT3, SIRT6, SIRT7 |
Class: Class III HDACs (Sirtuins) Function:
|
ING2 | Function: Inhibitor of Growth 2 (ING2) is not an HDAC but rather a protein that interacts with histone acetyltransferases (HATs) and histone methyltransferases (HMTs). ING2 acts as a tumor suppressor and is involved in modulating chromatin structure, DNA repair, and apoptosis. |
RBBP4 (Retinoblastoma-binding protein 4) and RBBP7 | Function: RBBP4 and RBBP7, also known as RbAp46 and RbAp48, respectively, are not HDACs but are associated with various chromatin-modifying complexes. They are involved in histone binding, nucleosome assembly, and transcriptional regulation. RBBP4 and RBBP7 often interact with HDACs and contribute to their functions. |
Understanding their functions helps in unraveling the complex mechanisms of gene regulation and provides insights into their potential therapeutic applications in diseases related to dysregulated gene expression.
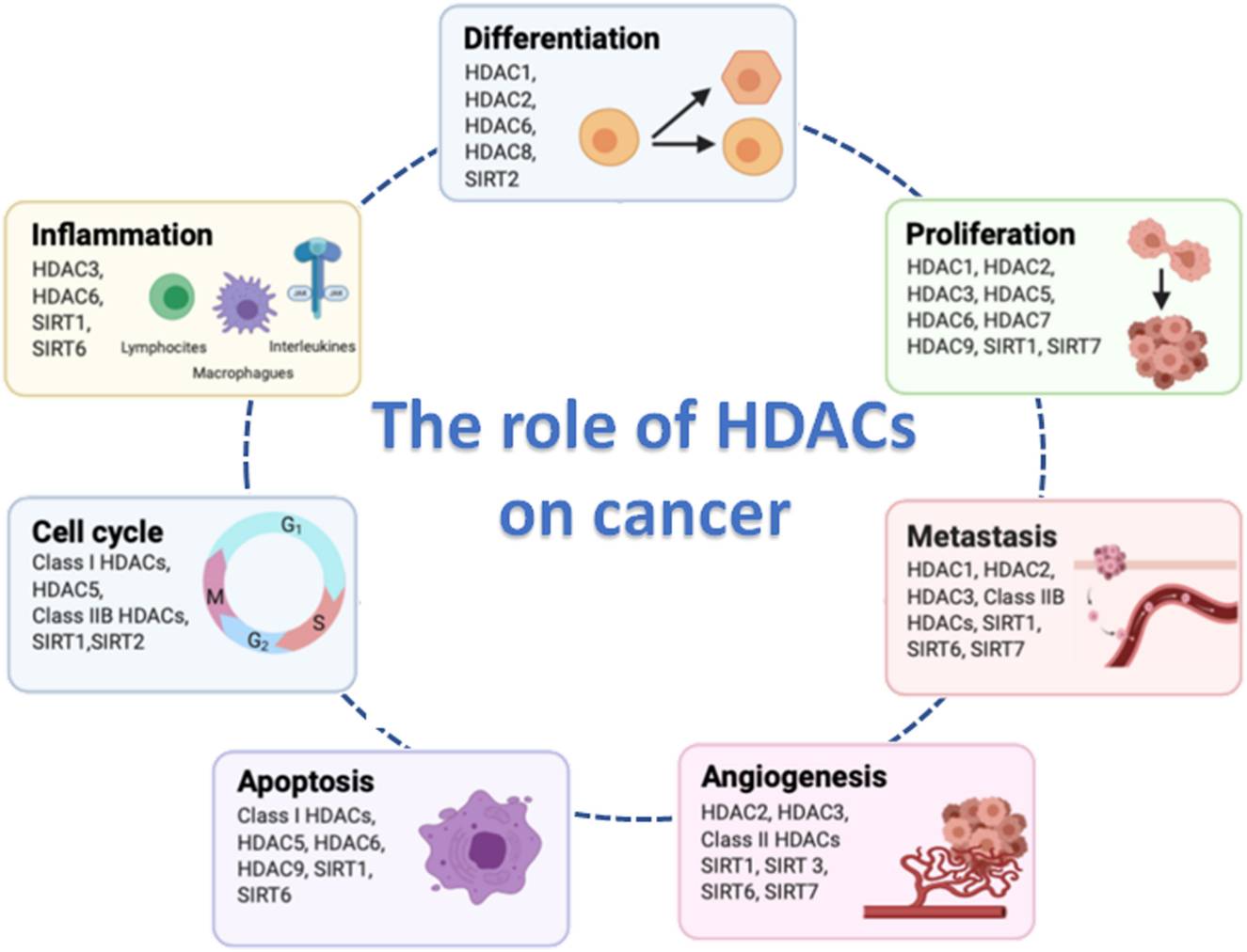
Regulation of HDAC Activity
HDAC activity is tightly regulated by various factors, including post-translational modifications, protein-protein interactions, and subcellular localization. Here's a description of these regulatory mechanisms:
Regulation Mechanism | Details |
---|---|
Post-Translational Modifications |
|
Protein-Protein Interactions |
HDACs interact with various proteins that can influence their activity. Examples include:
|
Subcellular Localization |
The subcellular localization of HDACs is crucial for their function and regulation. HDACs shuttle between the nucleus and the cytoplasm, and their localization can be influenced by various factors, such as:
|
Covalent Modifications of Histones |
|
These regulatory mechanisms collectively control HDAC expression, enzymatic activity, and their association with specific genomic loci. Dysregulation of these processes can lead to aberrant HDAC activity and contribute to disease pathogenesis.
It's worth noting that the regulation of HDACs is complex, involving multiple layers of control and interactions with various cellular components. Further research is needed to fully understand the intricacies of HDAC regulation and its implications in different physiological and pathological contexts.
Role of HDACs in Diseases
Cancer
Aberrant HDAC activity is frequently observed in various types of cancer, contributing to tumor initiation, progression, and resistance to therapy. Examples include:
- HDAC1 and HDAC2 overexpression in colorectal cancer, which leads to the repression of tumor suppressor genes like p21 and contributes to uncontrolled cell growth.
- HDAC6 upregulation in breast cancer, promoting cancer cell migration, invasion, and metastasis.
- HDAC inhibitors, such as vorinostat and romidepsin, showing efficacy in the treatment of cutaneous T-cell lymphoma and multiple myeloma.
Neurodegenerative Disorders
Aberrant HDAC activity has been linked to neurodegenerative diseases characterized by protein misfolding and aggregation. Examples include:
- HDAC6 involvement in the clearance of misfolded proteins in neurodegenerative disorders like Alzheimer's and Parkinson's diseases.
- HDAC inhibition showing neuroprotective effects in preclinical models of Huntington's disease, reducing aggregate formation and improving motor function.
- HDAC2 dysregulation in Alzheimer's disease, contributing to cognitive decline and synaptic dysfunction.
Inflammatory Conditions
Abnormal HDAC activity has been associated with inflammatory conditions, where it can regulate immune responses and inflammatory gene expression. Examples include:
- HDAC3 playing a role in rheumatoid arthritis by promoting inflammation and joint destruction.
- HDAC inhibitors, such as trichostatin A and suberoylanilide hydroxamic acid (SAHA), exhibiting anti-inflammatory effects by suppressing pro-inflammatory cytokines in models of asthma and inflammatory bowel disease.
- HDAC6 involvement in regulating inflammatory responses in sepsis and acute lung injury.
Other Diseases
Aberrant HDAC activity has also been implicated in other diseases, including:
- Cardiovascular diseases: HDAC inhibition showing cardioprotective effects by attenuating cardiac hypertrophy and fibrosis in models of heart failure.
- Diabetes: HDAC1 and HDAC3 dysregulation contributing to impaired insulin signaling and glucose metabolism.
- Psychiatric disorders: Altered HDAC activity observed in depression and schizophrenia, affecting synaptic plasticity and gene expression regulation.
These examples highlight the diverse roles of HDACs in disease pathogenesis and the potential of targeting HDAC activity for therapeutic intervention. However, it's important to note that the precise mechanisms and contributions of specific HDACs may vary across different disease contexts, and further research is needed to fully understand their roles and develop effective treatments.
Case Study
Case 1: Travers JG, Wennersten SA, Peña B, et al. HDAC inhibition reverses preexisting diastolic dysfunction and blocks covert extracellular matrix remodeling. Circulation. 2021;143(19):1874-1890.
Diastolic dysfunction (DD) is a condition associated with heart failure and other cardiac disorders. Histone deacetylase (HDAC) inhibition has shown promise in preventing DD by improving myofibril relaxation. In this study, the therapeutic potential of HDAC inhibition was investigated in a model of established DD with preserved ejection fraction.
The effects of HDAC inhibition on fibroblast activation were assessed in rat and human cardiac fibroblasts stimulated with TGF-β, which induces a myofibroblast phenotype. Treatment with the HDAC inhibitor ITF2357/Givinostat reduced the expression of α-SMA (a marker of myofibroblast activation) and stress fiber formation in stimulated fibroblasts. Fibronectin expression, necessary for collagen network deposition, was also reduced. ITF2357/Givinostat inhibited α-SMA protein expression and attenuated the induction of Acta2 (α-SMA) and Fn1 (fibronectin-1) transcripts.
Additionally, the ability of activated fibroblasts to contract collagen gels was evaluated. HDAC inhibition significantly reduced both basal and TGF-β-induced collagen gel contraction in rat and human cardiac fibroblasts over a 72-hour period.
Similar results were observed in normal human ventricular fibroblasts, further supporting the potential translatability of these findings to humans. HDAC inhibitor treatment attenuated TGF-β-induced α-SMA expression, stress fiber formation, and collagen gel contraction in human cardiac fibroblasts.
These findings demonstrate the role of HDACs in controlling cardiac fibroblast activation and suggest the potential of HDAC inhibitors in blocking cardiac fibrosis in humans.
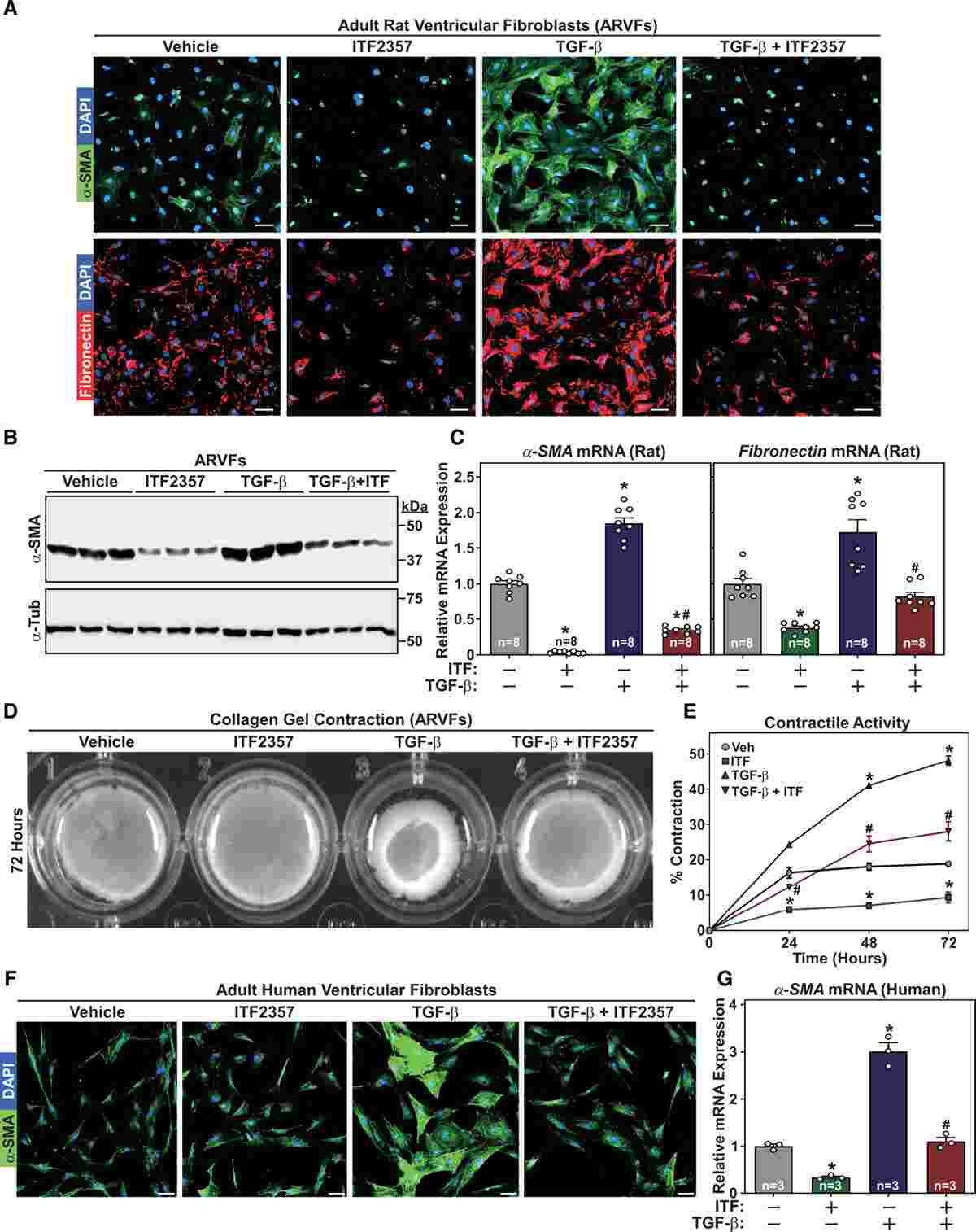
Case 2: Jung JK, Yoon GE, Jang G, Park KM, Kim I, Kim JI. Inhibition of HDACs (histone deacetylases) ameliorates high-fat diet-induced hypertension through restoration of the MsrA (methionine sulfoxide reductase A)/hydrogen sulfide axis. Hypertension. 2021;78(4):1103-1115.
This study investigated the effects of HDAC inhibition on the production of endogenous hydrogen sulfide (H2S) and hypertension. The researchers focused on the role of methionine sulfoxide reductase A (MsrA) in high-fat diet (HFD)-induced hypertension and examined the impact of HDAC inhibition on MsrA expression, H2S production, and hypertension.
They found that HFD increased the levels of oxidative stress markers and proinflammatory factors in the MAs (mesenteric arteries). However, treatment with the HDAC inhibitor CG200745 reversed these effects. HFD also upregulated the expression of MLCK, phospho-MYPT1, and phospho-MLC20, which are associated with increased contractility of MAs. Again, CG200745 administration significantly reversed the effects of HFD on these proteins.
In conclusion, HDAC inhibition with CG200745 showed beneficial effects in HFD-induced hypertension, reducing oxidative stress, inflammation, and improving MAs contractility. These findings suggest a potential involvement of HDACs and MsrA in H2S production and hypertension regulation.
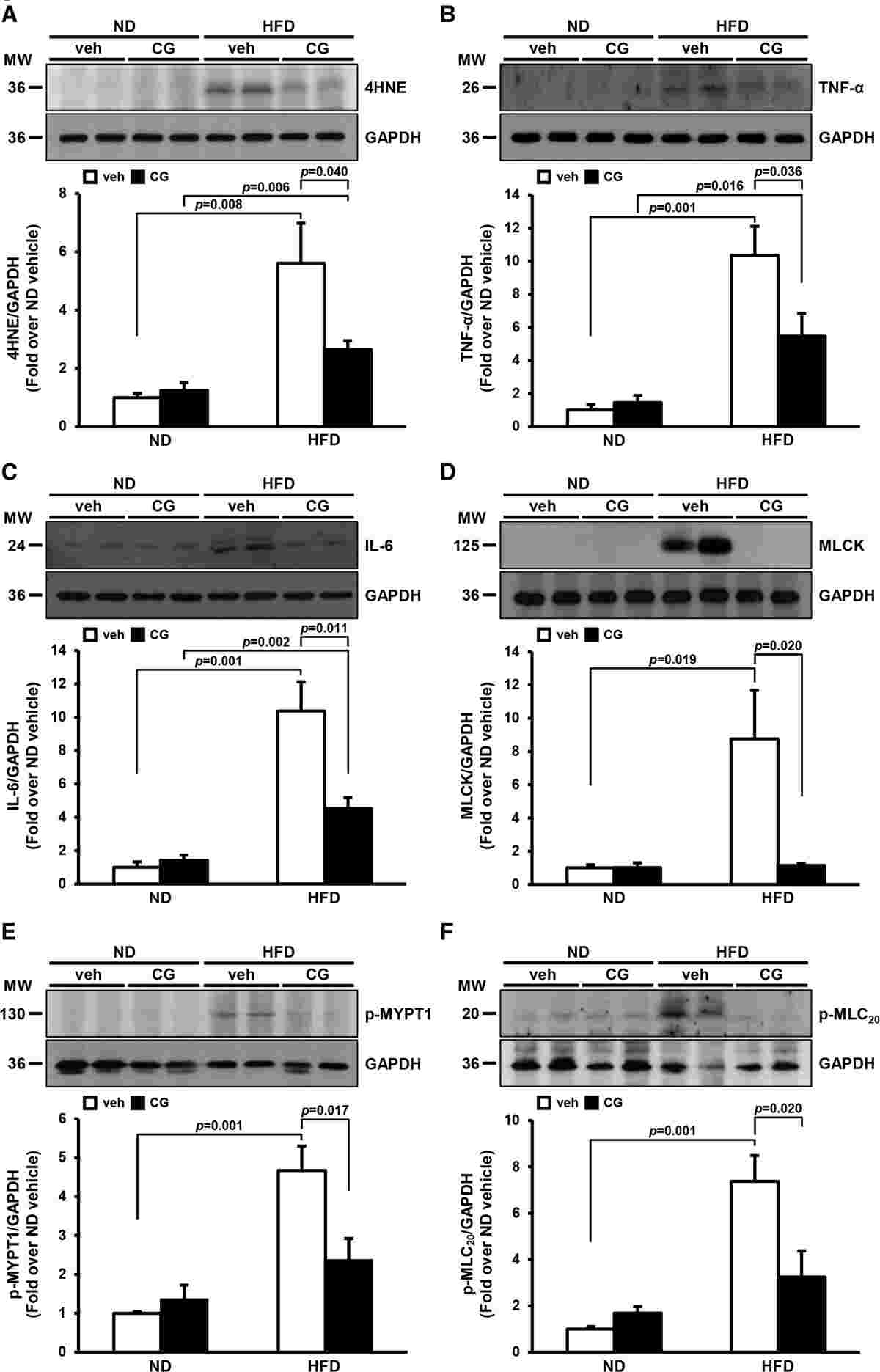
Related References
- Seto E, Yoshida M. Erasers of histone acetylation: the histone deacetylase enzymes. Cold Spring Harb Perspect Biol. 2014;6(4):a018713.
- Park SY, Kim JS. A short guide to histone deacetylases including recent progress on class II enzymes. Exp Mol Med. 2020;52(2):204-212.
- Milazzo G, Mercatelli D, Di Muzio G, et al. Histone deacetylases (HDACs): evolution, specificity, role in transcriptional complexes, and pharmacological actionability. Genes (Basel). 2020;11(5):556.
- Hontecillas-Prieto L, Flores-Campos R, Silver A, de Álava E, Hajji N, García-Domínguez DJ. Synergistic enhancement of cancer therapy using HDAC inhibitors: opportunity for clinical trials. Front Genet. 2020;11:578011.