Cell Metabolism
Creative BioMart Cell Metabolism Product List
Immunology Background
Background
Cellular metabolism involves the complex network of biochemical reactions that occur in living organisms to sustain life. These processes allow cells to grow, divide, produce energy, repair themselves, and respond to their environment. Metabolism is divided into two main categories: catabolism, which breaks down molecules to produce energy, and anabolism, which builds larger molecules from smaller ones. Understanding the intricacies of cellular metabolism is critical because it not only provides insight into normal physiological processes, but also reveals the biochemical underpinnings of diseases such as cancer.
Catabolism and Anabolism
Cell metabolism refers to the totality of biochemical reactions that occur within cells to convert nutrients into energy and building blocks necessary for cellular function. Metabolism is essential for sustaining life and involves a vast array of chemical transformations carried out by enzymes that facilitate the reactions at a rate sufficient to meet the demands of the cell.
Metabolic reactions can be broadly divided into two types:
Catabolic Pathways: These pathways break down complex molecules, such as carbohydrates, fats, and proteins, into simpler molecules such as carbon dioxide and water. The breakdown releases energy, primarily in the form of adenosine triphosphate (ATP), the universal energy currency of the cell.
Anabolic Pathways: These pathways use energy to synthesize complex molecules from simpler ones. Examples include the synthesis of proteins from amino acids and nucleic acids from nucleotides. Anabolism is essential for growth, tissue repair, and reproduction.
Both catabolism and anabolism are tightly regulated and interconnected, ensuring that cells efficiently use resources, maintain energy homeostasis, and respond to internal and external signals.
Main Steps of Cell Metabolism
Cell metabolism involves several key steps that vary depending on the type of nutrient being metabolized. The major pathways include glycolysis, the citric acid cycle (Krebs cycle), oxidative phosphorylation, and lipid metabolism.
Glycolysis
Glycolysis is the first step in the metabolism of glucose, the most abundant source of energy for cells. It occurs in the cytoplasm and does not require oxygen, making it the primary pathway for energy production in both aerobic and anaerobic conditions. During glycolysis, one molecule of glucose (a six-carbon sugar) is broken down into two molecules of pyruvate (a three-carbon compound), producing a net gain of two ATP molecules and two molecules of nicotinamide adenine dinucleotide (NADH), an electron carrier.
Key steps in glycolysis include:
- Glucose phosphorylation by hexokinase to form glucose-6-phosphate.
- Conversion of glucose-6-phosphate to fructose-6-phosphate.
- Fructose-6-phosphate is phosphorylated to fructose-1,6-bisphosphate, catalyzed by phosphofructokinase (a key regulatory step).
- The splitting of fructose-1,6-bisphosphate into two three-carbon molecules, glyceraldehyde-3-phosphate and dihydroxyacetone phosphate.
- Pyruvate formation, yielding ATP and NADH.
In the presence of oxygen, pyruvate enters the mitochondria for further oxidation in the citric acid cycle. In the absence of oxygen, pyruvate is converted into lactate through anaerobic glycolysis.
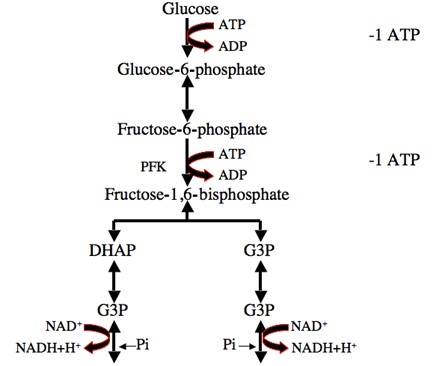
Citric Acid Cycle (Krebs Cycle)
The citric acid cycle, also known as the Krebs cycle or tricarboxylic acid (TCA) cycle, takes place in the mitochondria. This aerobic process oxidizes the acetyl-CoA derived from pyruvate, fats, and amino acids to produce ATP, NADH, and flavin adenine dinucleotide (FADH2), another electron carrier.
Key steps in citric acid cycle include:
- Formation of Citrate: The cycle starts when acetyl-CoA combines with oxaloacetate to produce citrate, a six-carbon compound.
- Isomerization of Citrate: Citrate is then rearranged to form isocitrate through the action of aconitase.
- Oxidation and Decarboxylation: Isocitrate undergoes oxidation and is converted into α-ketoglutarate by the enzyme isocitrate dehydrogenase. This reaction generates NADH and releases carbon dioxide.
- Further Oxidation: α-Ketoglutarate is further oxidized to succinyl-CoA, catalyzed by α-ketoglutarate dehydrogenase. This step also produces NADH and releases another molecule of carbon dioxide.
- Substrate-Level Phosphorylation: Succinyl-CoA is transformed into succinate, resulting in the production of ATP (or GTP) through substrate-level phosphorylation, with the help of succinyl-CoA synthetase.
- Oxidation of Succinate: Succinate is then oxidized to fumarate by succinate dehydrogenase, generating FADH₂ in the process.
- Hydration of Fumarate: Fumarate is hydrated to form malate, facilitated by the enzyme fumarase, which adds a water molecule.
- Final Oxidation: Finally, malate is oxidized back to oxaloacetate by malate dehydrogenase, producing another molecule of NADH.
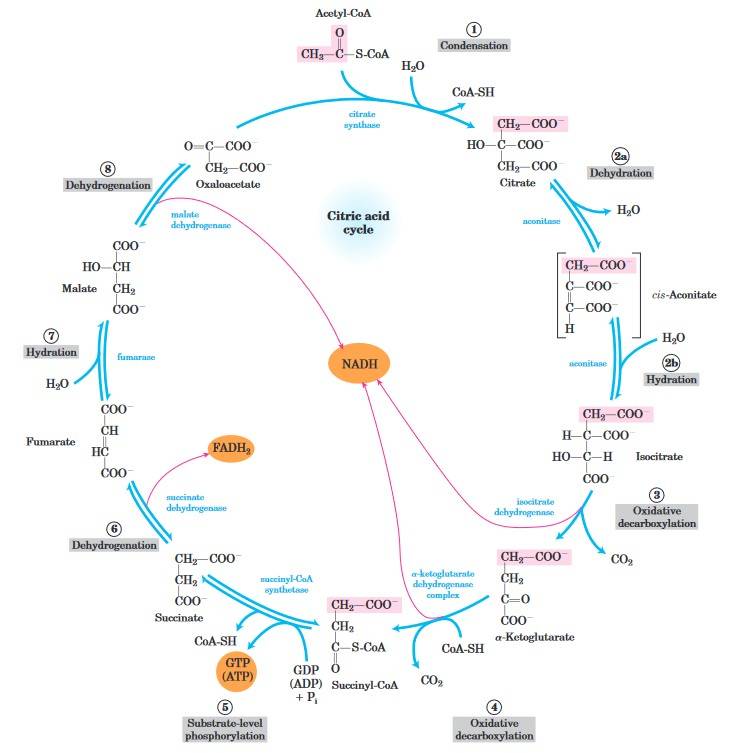
Oxidative Phosphorylation (Electron Transport Chain)
Oxidative phosphorylation is the process by which the energy stored in NADH and FADH2 is used to produce ATP. This process takes place in the inner mitochondrial membrane where the electron transport chain (ETC) is located. As electrons are transferred through a series of complexes (I-IV) in the ETC, protons are pumped across the inner mitochondrial membrane, creating an electrochemical gradient. This gradient drives ATP synthesis by ATP synthase, a process known as chemiosmosis. Each NADH molecule produces about three molecules of ATP, while each FADH2 molecule produces two molecules of ATP. Oxygen acts as the final electron acceptor, combining with protons to form water.
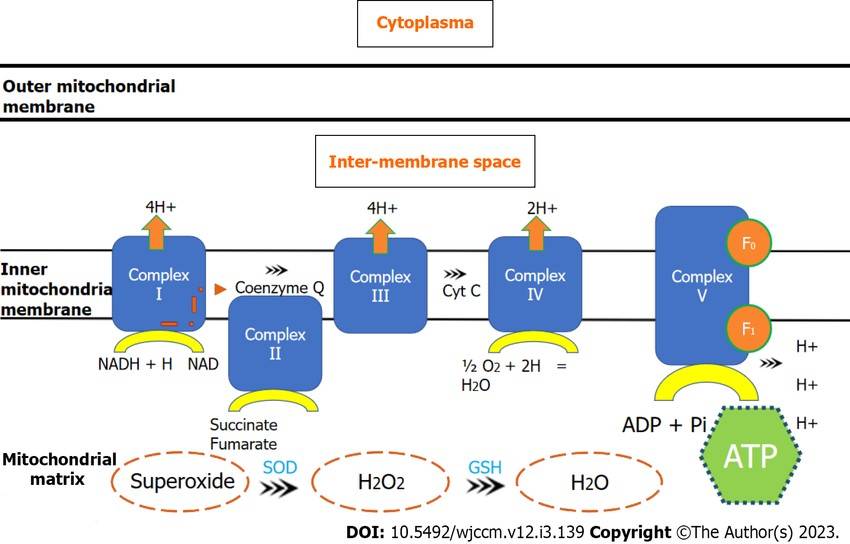
Lipid Metabolism
Lipid metabolism is another critical pathway, especially in tissues such as liver and muscle, where fatty acids are used as an energy source. Fatty acids undergo β-oxidation in the mitochondria, which breaks them down into acetyl-CoA molecules. These acetyl-CoA molecules then enter the citric acid cycle for further oxidation. Fat metabolism yields significantly more ATP than glucose metabolism, making it an important energy reserve during fasting or prolonged exercise.
Control and Regulation of Cellular Metabolism
The regulation of cellular metabolism is complex and involves a combination of genetic, hormonal and environmental signals. Several key factors and signaling pathways control metabolism to ensure that the cell's energy needs are met and metabolic waste is efficiently disposed of.
Enzymatic Control
Enzymes are at the core of metabolic regulation. These proteins catalyze metabolic reactions, and their activity can be modulated by several mechanisms, including allosteric regulation, covalent modification (e.g., phosphorylation), and changes in enzyme synthesis or degradation. For example, phosphofructokinase, the enzyme that regulates glycolysis, is allosterically activated by AMP, indicating low energy levels, and inhibited by ATP and citrate, signaling that the cell has sufficient energy.
Genetic Regulation
Gene expression plays an important role in the regulation of metabolism. Certain genes encode metabolic enzymes and transport proteins, and their expression can be upregulated or downregulated in response to changes in nutrient availability or hormonal signals. For example, during fasting, the expression of enzymes involved in gluconeogenesis (the synthesis of glucose) is upregulated in the liver, which supplies glucose to the brain and other organs.
Hormonal Control
Hormones are key regulators of metabolism, coordinating metabolic activities in different tissues. Insulin and glucagon, secreted by the pancreas, are the primary hormones involved in maintaining glucose homeostasis. Insulin promotes the uptake of glucose by cells and stimulates anabolic processes such as glycogenesis (the synthesis of glycogen) and lipogenesis (the synthesis of fats). Glucagon, on the other hand, promotes catabolic processes such as glycogenolysis (the breakdown of glycogen) and gluconeogenesis during fasting.
Other hormones involved in metabolic regulation include:
- Thyroid hormones: Regulate basal metabolic rate by increasing the activity of mitochondrial enzymes involved in oxidative phosphorylation.
- Adrenaline (epinephrine): Stimulates glycogenolysis and lipolysis, providing fuel during the "fight or flight" response.
- Cortisol: Increases glucose production through gluconeogenesis and promotes fat metabolism during prolonged stress.
Nutrient Availability and Energy Sensing Pathways
Cells can sense the availability of nutrients and energy through specific signaling pathways. One of the most well-known pathways is the AMPK (AMP-activated protein kinase) pathway. AMPK is activated when cellular energy levels are low, as indicated by an increase in AMP levels. Once activated, AMPK promotes catabolic pathways that generate ATP and inhibits anabolic pathways to conserve energy.
The mTOR (mechanistic target of rapamycin) pathway is another critical regulator of metabolism, particularly in the context of nutrient availability. mTOR promotes cell growth and protein synthesis in response to nutrients, growth factors, and energy availability. Dysregulation of the mTOR pathway is implicated in various diseases, including cancer.
Association with Cancer
Metabolic dysregulation has emerged as a defining characteristic of cancer cells, often referred to as the hallmark of cancer. Cancer cells undergo profound changes in their metabolism to fuel the rapid proliferation, migration, and survival necessary for tumor progression. This reprogramming of cellular metabolism, widely known as the "Warburg effect," highlights the unique metabolic needs of cancer cells and represents a paradigm shift in how scientists view cancer biology. Otto Warburg first described this phenomenon in the 1920s, showing that cancer cells tend to favor glycolysis even when oxygen is abundantly available, which is atypical of normal cellular metabolism.
The Warburg Effect
One of the most striking and defining metabolic changes in cancer cells is their increased reliance on glycolysis, even in the presence of oxygen, a condition referred to as aerobic glycolysis. In healthy cells, oxidative phosphorylation, which occurs in the mitochondria, is the preferred and most efficient pathway for ATP production in the presence of oxygen. Oxidative phosphorylation produces approximately 36 molecules of ATP per molecule of glucose, making it far more efficient than glycolysis, which produces only two molecules of ATP per molecule of glucose. However, cancer cells tend to favor glycolysis over oxidative phosphorylation, even though glycolysis produces significantly less ATP.
This shift to glycolysis allows cancer cells to maintain high levels of ATP and intermediates necessary for anabolic processes, such as nucleotide, amino acid, and lipid biosynthesis, in the presence of sufficient oxygen, which is critical to support the rapid proliferation of tumor cells. Although less energetically efficient, aerobic glycolysis provides cancer cells with additional advantages that promote tumor growth and survival.
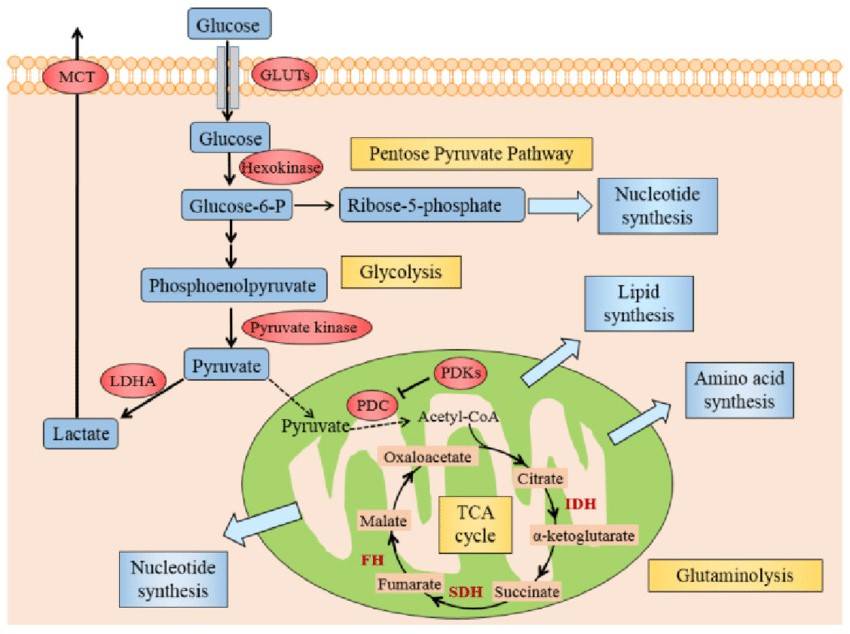
Cancer Metabolism as a Therapeutic Target
The dependence of cancer cells on altered metabolic pathways, particularly glycolysis, has prompted the development of therapeutic strategies aimed at targeting these unique metabolic vulnerabilities. Disrupting the metabolic adaptations on which cancer cells depend represents an attractive approach to cancer therapy. Several metabolic pathways have emerged as potential targets for cancer therapy:
- Inhibiting Glycolysis: Blocking glycolysis, a primary source of ATP for many cancer cells, with 2-deoxy-D-glucose (2-DG) reduces proliferation and improves therapy, although human efficacy is still being studied.
- Targeting the mTOR Pathway: mTOR inhibitors such as rapamycin inhibit cancer cell growth by blocking anabolic processes, but may require combination therapies to overcome resistance.
- Targeting Fatty Acid Metabolism: Inhibition of lipid metabolism enzymes such as acetyl-CoA carboxylase (ACC) and fatty acid synthase (FASN) disrupts energy production and membrane synthesis in cancers dependent on fatty acid metabolism.
- Metformin and AMPK Activation: Metformin activates AMPK, which suppresses anabolic pathways and inhibits mTOR signaling, potentially reducing cancer growth and improving outcomes.
- Lactate as a Therapeutic Target: Inhibiting lactate dehydrogenase (LDH) to reduce lactate production may counteract tumor acidity and enhance immune-based cancer therapies.
- Immunometabolism: Targeting metabolic enzymes such as indoleamine 2,3-dioxygenase (IDO) prevents tumor immune suppression and enhances anti-tumor immune responses, with promising results in clinical trials.
In conclusion, cell metabolism is a complex and highly regulated network of biochemical reactions essential for life. It involves several key steps, including glycolysis, the citric acid cycle, oxidative phosphorylation, and lipid metabolism, all of which are controlled by enzymes, gene expression, hormones, and nutrient-sensing pathways. Dysregulation of cellular metabolism is a hallmark of cancer, with cancer cells exhibiting metabolic reprogramming to support their rapid growth and survival. Understanding the intricacies of metabolism and its role in disease provides valuable insights into potential therapeutic targets for cancer and other metabolic disorders.
Case Study
Case 1: Kim, C.-W.; et al. Acetyl CoA carboxylase inhibition reduces hepatic steatosis but elevates plasma triglycerides in mice and humans: A bedside to bench investigation. Cell Metabolism. 2017, 26(2), 394-406.e6.
Kim et al. report on an acetyl-CoA carboxylase (ACC) 1 and 2 inhibitor MK-4074 that reduces hepatic triglycerides in individuals with fatty liver but increases plasma triglyceride levels. In ACC-deficient mice, reduced malonyl-CoA levels suppress polyunsaturated fatty acid synthesis, which triggers increased expression of SREBP-1c and GPAT1, leading to increased VLDL secretion and hypertriglyceridemia.
Phase 1 data showed that MK-4074 effectively inhibited ACC1 and ACC2 in humans. A pioglitazone arm was included for comparison as pioglitazone is known to reduce liver triglycerides and fibrosis. Hepatic fat content was measured by MRI before treatment and after 4 weeks of treatment.
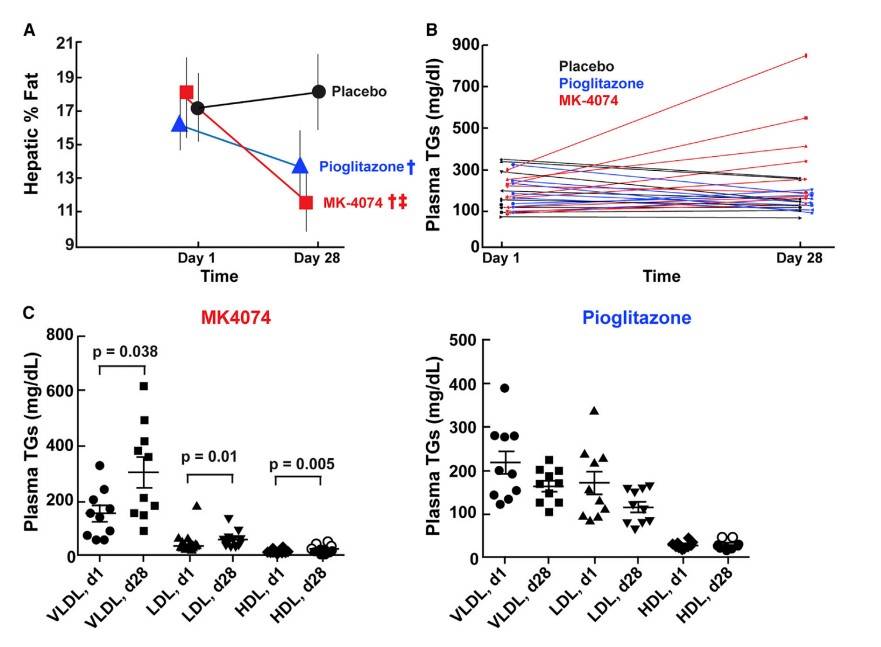
Case 2: Lu, W.; et al. Novel role of NOX in supporting aerobic glycolysis in cancer cells with mitochondrial dysfunction and as a potential target for cancer therapy. PLoS Biology. 2012, 10(5), e1001326.
Increased aerobic glycolysis in cancer cells (the Warburg effect) has been associated with mitochondrial dysfunction or respiratory injury, although the mechanisms and therapeutic relevance remain unclear. The Lu group discovered that NOX upregulation is essential to support increased glycolysis by providing additional NAD+. This upregulation is also observed in cancer cells with mitochondrial impairment, such as those with oncogenic Ras activation or p53 loss, and in primary pancreatic cancer tissues. Inhibition of NOX, either by chemical or genetic knockdown, selectively affects cancer cells with mitochondrial dysfunction, reducing glycolysis, cell viability and tumor growth in vivo. This study uncovers a novel role for NOX in cancer metabolism and highlights it as a promising target for cancer therapy.
They investigated the role of NOX in glucose metabolism by assessing the effects of NOX knockdown on glucose uptake, ATP production, and NAD+ levels. Using siRNA, they specifically targeted NOX1 and p22phox (an essential component of phagocyte NADPH oxidase) in both doxycycline-induced (Tet/on) and doxycycline-repressed (Tet/off) cells. Notably, NOX silencing by siRNA selectively affected cells with mitochondrial dysfunction (Tet/on), resulting in a significant reduction in glucose uptake and ATP production. In contrast, cells with intact mitochondrial function (Tet/off) were unaffected, suggesting that NOX plays a key role in glucose metabolism in highly glycolytic cells, such as those with mitochondrial defects.
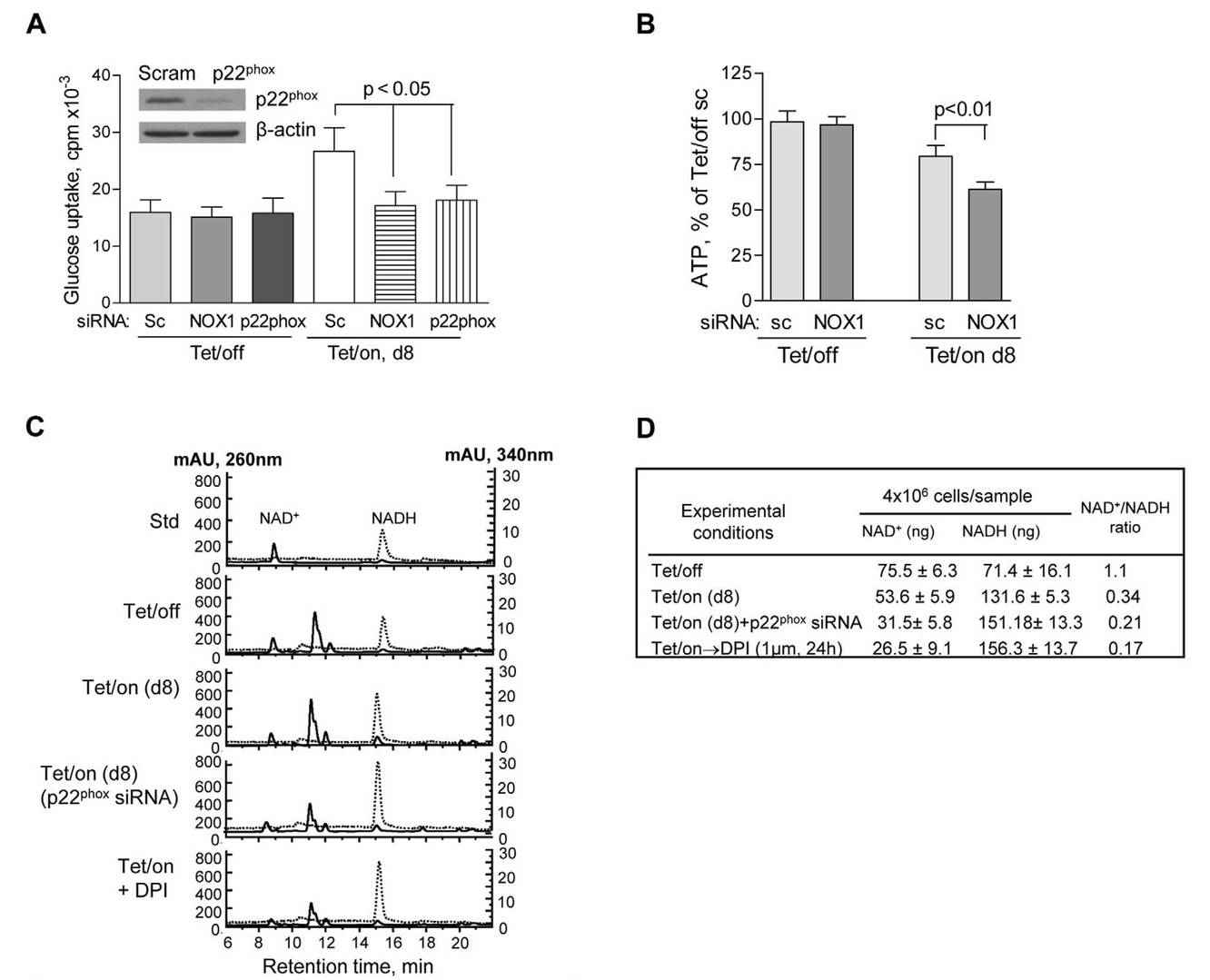
References
- Lehninger Principles of Biochemistry Sixth Edition, 2013.
- Aghaee, F., Pirayesh Islamian, J., & Baradaran, B. (2012). Enhanced radiosensitivity and chemosensitivity of breast cancer cells by 2-deoxy-d-glucose in combination therapy. Journal of Breast Cancer, 15(2), 141.
- Atkins, M. B., Yasothan, U., & Kirkpatrick, P. (2009). Everolimus. Nature Reviews Drug Discovery, 8(7), 535–536.
- Hsu, S.-K., Cheng, K.-C., Mgbeahuruike, M. O., Lin, Y.-H., Wu, C.-Y., Wang, H.-M. D., Yen, C.-H., Chiu, C.-C., & Sheu, S.-J. (2021). New insight into the effects of metformin on diabetic retinopathy, aging and cancer: Nonapoptotic cell death, immunosuppression, and effects beyond the AMPK pathway. International Journal of Molecular Sciences, 22(17), 9453.
- Kim, C.-W., Addy, C., Kusunoki, J., Anderson, N. N., Deja, S., Fu, X., Burgess, S. C., Li, C., Ruddy, M., Chakravarthy, M., Previs, S., Milstein, S., Fitzgerald, K., Kelley, D. E., & Horton, J. D. (2017). Acetyl CoA carboxylase inhibition reduces hepatic steatosis but elevates plasma triglycerides in mice and humans: A bedside to bench investigation. Cell Metabolism, 26(2), 394-406.e6.
- Li, X., Yang, Y., Zhang, B., Lin, X., Fu, X., An, Y., Zou, Y., Wang, J.-X., Wang, Z., & Yu, T. (2022). Lactate metabolism in human health and disease. Signal Transduction and Targeted Therapy, 7(1), 1–22.
- Lu, W., Hu, Y., Chen, G., Chen, Z., Zhang, H., Wang, F., Feng, L., Pelicano, H., Wang, H., Keating, M. J., Liu, J., McKeehan, W., Wang, H., Luo, Y., & Huang, P. (2012). Novel role of nox in supporting aerobic glycolysis in cancer cells with mitochondrial dysfunction and as a potential target for cancer therapy. PLoS Biology, 10(5), e1001326.
- Nedel, W., Deutschendorf, C., & Portela, L. V. C. (2023). Sepsis-induced mitochondrial dysfunction: A narrative review. World Journal of Critical Care Medicine, 12(3), 139–152.
- Pernicova, I., & Korbonits, M. (2014). Metformin—Mode of action and clinical implications for diabetes and cancer. Nature Reviews Endocrinology, 10(3), 143–156.
- Wicker, C. A., Hunt, B. G., Krishnan, S., Aziz, K., Parajuli, S., Palackdharry, S., Elaban, W. R., Wise-Draper, T. M., Mills, G. B., Waltz, S. E., & Takiar, V. (2021). Glutaminase inhibition with telaglenastat (CB-839) improves treatment response in combination with ionizing radiation in head and neck squamous cell carcinoma models. Cancer Letters, 502, 180–188.
- Zhang, W., Zhang, S.-L., Hu, X., & Tam, K. Y. (2015). Targeting tumor metabolism for cancer treatment: Is pyruvate dehydrogenase kinases (PDKs) a viable anticancer target? International Journal of Biological Sciences, 11(12), 1390–1400.