Vascular Smooth Muscle
Creative BioMart Vascular Smooth Muscle Product List
Immunology Background
Background
Vascular smooth muscle cells (VSMC) are specialized contractile cells found in the walls of blood vessels that play a critical role in regulating vascular tone, blood flow, and blood pressure. Unlike cardiac and skeletal muscle, VSMC are non-striated and have unique properties that allow them to adapt to various physiological and pathological conditions.
Structure of Vascular Smooth Muscle
Vascular smooth muscle cells are elongated, spindle-shaped cells that are interconnected by gap junctions, allowing for coordinated contraction. They are found primarily in the media of blood vessels, between the endothelium and the outer adventitia. The number of layers of smooth muscle cells varies with the type of blood vessel. In larger arteries, such as the aorta, multiple layers of smooth muscle cells are present, while smaller arterioles may consist of a single layer.
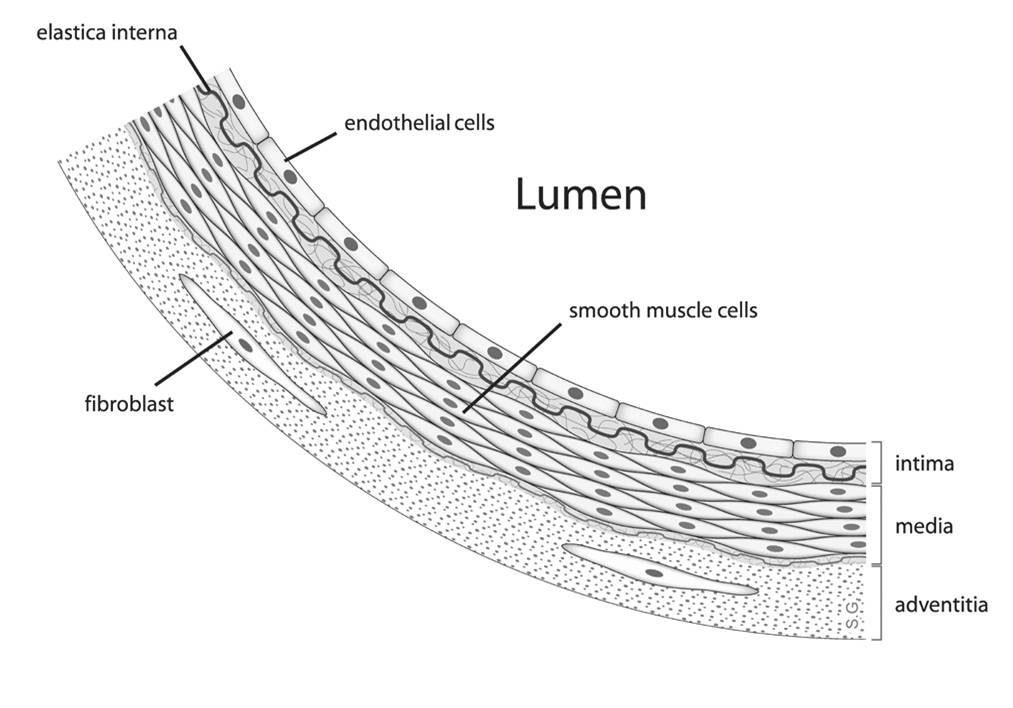
Vascular smooth muscle cells are traditionally described as fusiform cells with a prominent central nucleus surrounded by abundant endoplasmic reticulum and Golgi apparatus. The cytoplasm of VSMCs contains a dense array of contractile filaments composed of actin and myosin organized into a mesh-like structure. This organization facilitates contraction in response to various stimuli. VSMC also contain organelles such as rough and smooth endoplasmic reticulum, Golgi apparatus, and mitochondria that support their metabolic and synthetic functions. The cytoplasm and plasma membrane of VSMC taper toward the ends of the cell. Despite this narrowing, there is substantial evidence that the junctions connecting smooth muscle cells are complex, with numerous membrane invaginations that increase the surface area for both mechanical tight junctions and electrical coupling through gap junctions. Unlike striated muscle, VSMC lack an elaborate T-tubule/sarcoplasmic reticulum system. Instead, they possess numerous plasma membrane invaginations called caveolae, which function similarly by increasing the surface-to-volume ratio of the cell. These caveolae create a specialized membrane environment that clusters specific ion channels and receptors, which play a key role in cellular signaling.
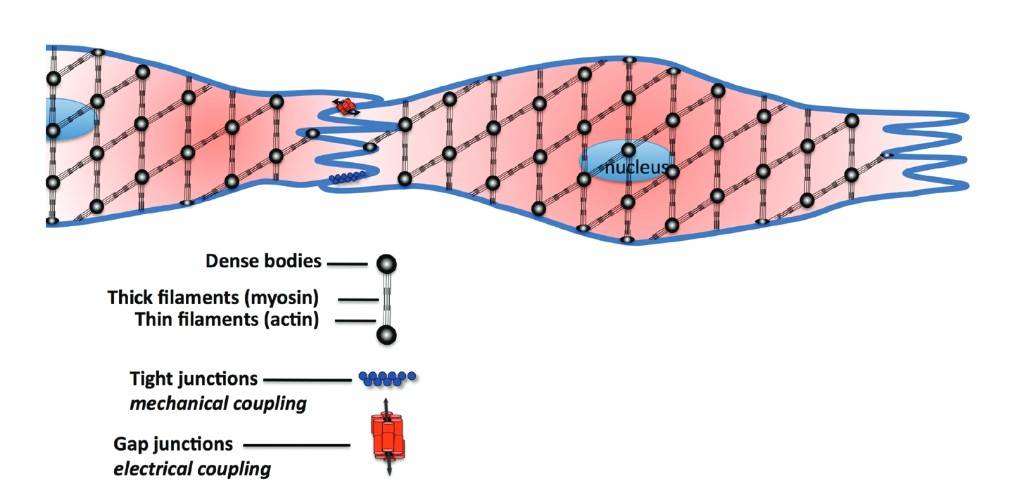
Vascular smooth muscle is surrounded by an extracellular matrix (ECM) composed of collagen, elastin, and glycoproteins. The ECM provides structural support and regulates VSMC behavior through biochemical signaling. The interaction between VSMC and ECM is critical for maintaining vascular integrity and function.
Functions of Vascular Smooth Muscle
Regulation of Vascular Tone
One of the primary functions of vascular smooth muscle is to regulate vascular tone, which is the degree of contraction of the smooth muscle surrounding blood vessels. Contraction of VSMCs results in vasoconstriction, which decreases the diameter of the blood vessel and increases blood pressure, while relaxation results in vasodilation, which increases the diameter of the blood vessel and decreases blood pressure. This regulation is critical for maintaining adequate blood flow to organs and tissues.
VSMC contraction is mediated by several signaling pathways, primarily involving calcium ions. When vascular smooth muscle cells are stimulated, calcium ions enter the cytoplasm, leading to the activation of myosin light chain kinase (MLCK). MLCK phosphorylates the myosin light chain, allowing it to interact with actin and initiate contraction. Conversely, vasodilation occurs through activation of myosin light chain phosphatase, which dephosphorylates myosin and promotes relaxation.
Response to Hormonal and Local Factors
Vascular smooth muscle cells respond to a variety of hormonal and local factors that modulate their activity. For example, norepinephrine released from sympathetic nerves causes vasoconstriction, while substances such as nitric oxide (NO) released from endothelial cells promote vasodilation. Other factors, such as prostacyclin and endothelin, also play important roles in regulating VSMC tone.
VSMC have receptors for a variety of signaling molecules, including hormones, neurotransmitters, and growth factors. Activation of these receptors triggers intracellular signaling cascades that regulate calcium levels and, subsequently, VSMC contraction or relaxation. This responsiveness allows the vasculature to adapt to changes in physiological demands, such as during exercise or in response to injury.
Role in Vascular Remodeling
Vascular smooth muscle cells are also involved in vascular remodeling, a process characterized by changes in the structure and function of blood vessels in response to various stimuli. VSMC can undergo phenotypic modulation, switching from a contractile phenotype to a synthetic phenotype in response to injury, inflammation, or increased hemodynamic stress.
In the synthetic phenotype, VSMC proliferate and secrete extracellular matrix components, contributing to the thickening of the vessel wall. This process is essential for healing after vascular injury, but can become maladaptive in conditions such as atherosclerosis, where excessive remodeling leads to vascular stiffness and luminal narrowing.
Pathophysiology of Vascular Smooth Muscle
Hypertension
Hypertension is a condition characterized by increased vascular resistance due to abnormal VSM function. In hypertensive states, VSMC exhibit increased contractility and decreased sensitivity to vasodilators, resulting in sustained vasoconstriction and increased peripheral resistance.
Chronic exposure to elevated pressure can induce structural changes in VSMC, including hypertrophy and hyperplasia, which contribute to the thickening of the vessel wall. This remodeling further exacerbates hypertension and increases the risk of cardiovascular events, including heart attack and stroke.
Atherosclerosis
As noted above, VSMC are integral to the development and progression of atherosclerosis. VSMC migration and proliferation in response to endothelial dysfunction are critical for plaque formation. However, excessive proliferation and matrix production can lead to plaque instability, increasing the risk of rupture and subsequent thrombus formation leading to acute cardiovascular events.
Inflammation is another critical factor in atherosclerosis, and VSMC contribute to the inflammatory milieu by producing pro-inflammatory cytokines and chemokines. This inflammatory milieu not only accelerates plaque development but also influences VSMC behavior, perpetuating the cycle of vascular dysfunction.
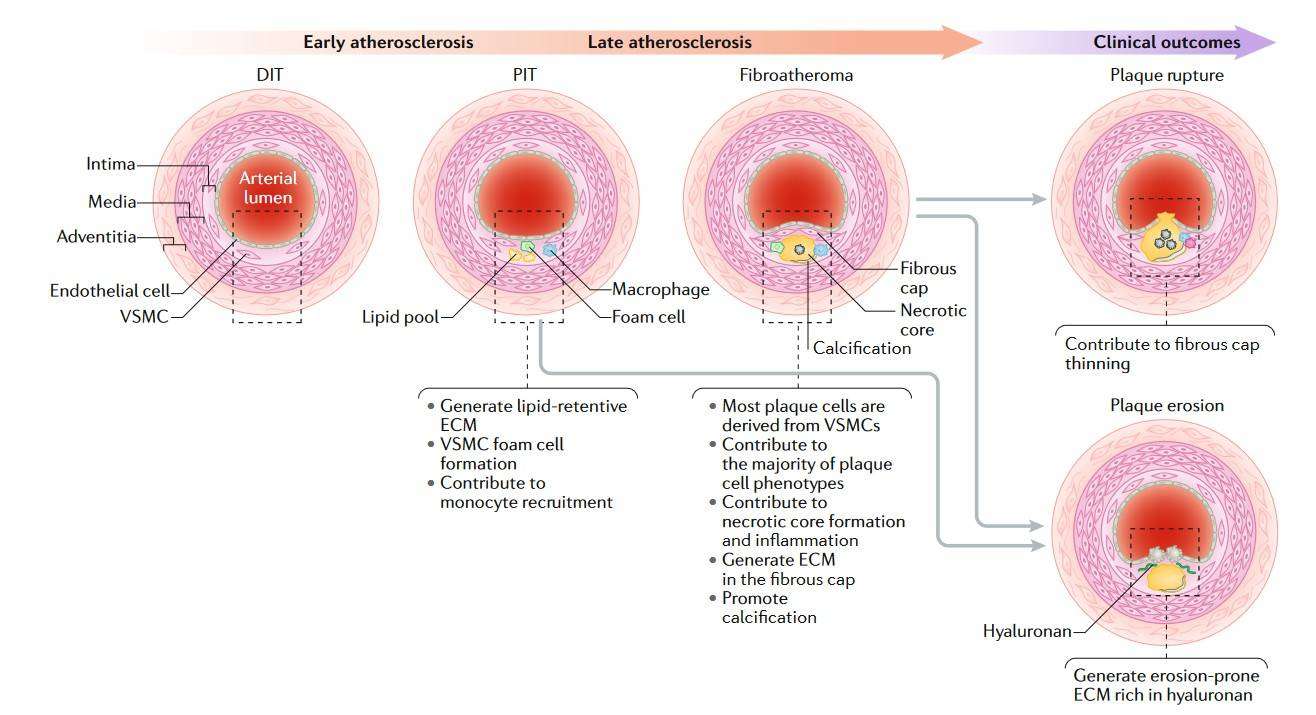
Vascular Remodeling in Diabetes
Diabetes mellitus is associated with significant alterations in vascular smooth muscle function. Hyperglycemia and increased levels of advanced glycation end products (AGEs) can lead to oxidative stress and inflammation, impairing VSM function and promoting vascular remodeling.
Diabetes-related vascular changes include increased vascular tone, decreased vasodilator responsiveness, and increased VSMC proliferation, which contribute to the development of diabetic complications such as peripheral artery disease and increased cardiovascular risk.
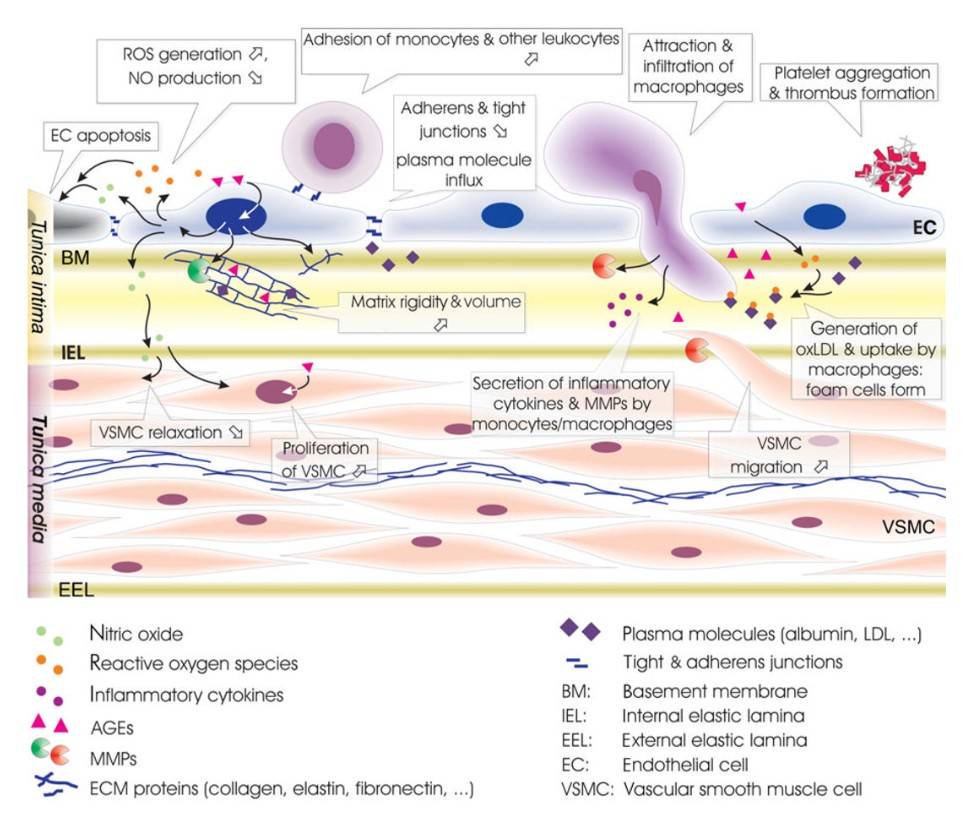
In summary, vascular smooth muscle cells are fundamental components of the vasculature and play critical roles in regulating vascular tone, responding to hormonal signals, participating in vascular remodeling, and contributing to the pathogenesis of cardiovascular disease. Understanding the structure and functions of VSMs, as well as the mechanisms underlying their dysregulation, is essential for the development of effective therapeutic strategies for the treatment of vascular diseases.
Case Study
Case 1: Allard, D.; et al. Akt regulates the survival of vascular smooth muscle cells via inhibition of Foxo3a and GSK3. Journal of Biological Chemistry. 2008, 283(28), 19739–19747.
This case study explores the molecular mechanisms by which IGF1R and Akt signaling regulate the survival of VSMCs in atherosclerotic plaques, focusing on the role of downstream targets FoxO3a and GSK3 in promoting survival and preventing apoptosis.
To determine which Akt targets support VSMC survival, the researchers examined the phosphorylation of potential substrates after activation of mAktER or A2AktER with HT or IGF1 in serum-deprived cells for 24 hours. Whereas Bad phosphorylation by Akt contributes to cell survival in BALB/c 3T3 cells, they found no evidence of Bad phosphorylation in VSMCs after Akt activation. However, Akt activation led to rapid phosphorylation of FoxO3a and both isoforms of GSK3 (α and β), an effect that was absent in A2AktER control cells. Similarly, IGF1 stimulation also resulted in phosphorylation of FoxO3a and GSK3, supporting the idea that Akt mediates signaling downstream of IGF1R in VSMCs. Interestingly, while FoxO3a was phosphorylated by Akt in VSMCs, other FoxO family members such as FoxO1 were not phosphorylated in response to HT or IGF1.
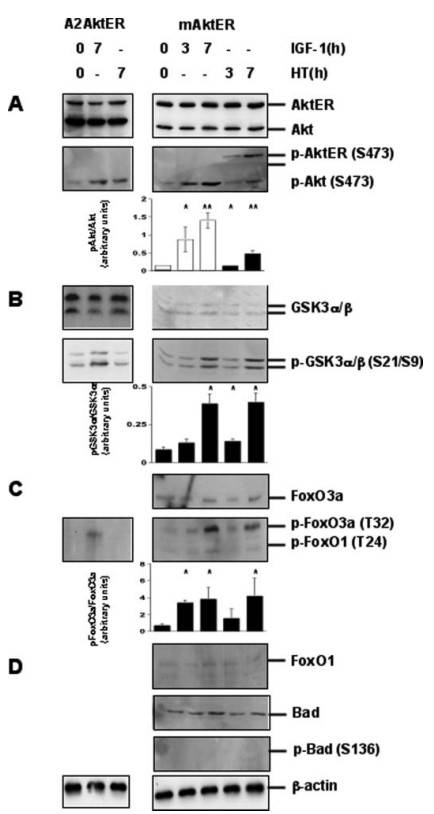
Case 2: Hwang, H. J.; et al. FLRT2 prevents endothelial cell senescence and vascular aging by regulating the ITGB4/mTORC2/p53 signaling pathway. JCI Insight. 2024, 9(7), e172678.
FLRT2 (fibronectin leucine-rich transmembrane protein 2) helps prevent endothelial cell senescence and vascular aging by regulating the ITGB4/mTORC2/p53 signaling pathway. Additionally, FLRT2 promotes monocyte differentiation into macrophages via the UNC5B-Akt/mTOR axis.
To explore the role of other β-integrins in senescence triggered by FLRT2 silencing, ITGB1, ITGB3, and ITGB5—known to be expressed in endothelial cells—were individually silenced. However, only silencing ITGB4 significantly suppressed the increase in mTORC2, AKT, p53, p21, and SA-β-Gal levels and activity caused by FLRT2 depletion, as well as the elevated expression of SASP factors IL-1α, IL-6, IL-8, and MMP3. Treatment with the ITGB4 inhibitor ASC-8 reduced AKT phosphorylation and the accumulation of p53 and p21 in FLRT2-silenced HUVECs, and decreased cell senescence. This indicates that ITGB4 plays a key role in the FLRT2 pathway that leads to endothelial cell senescence.
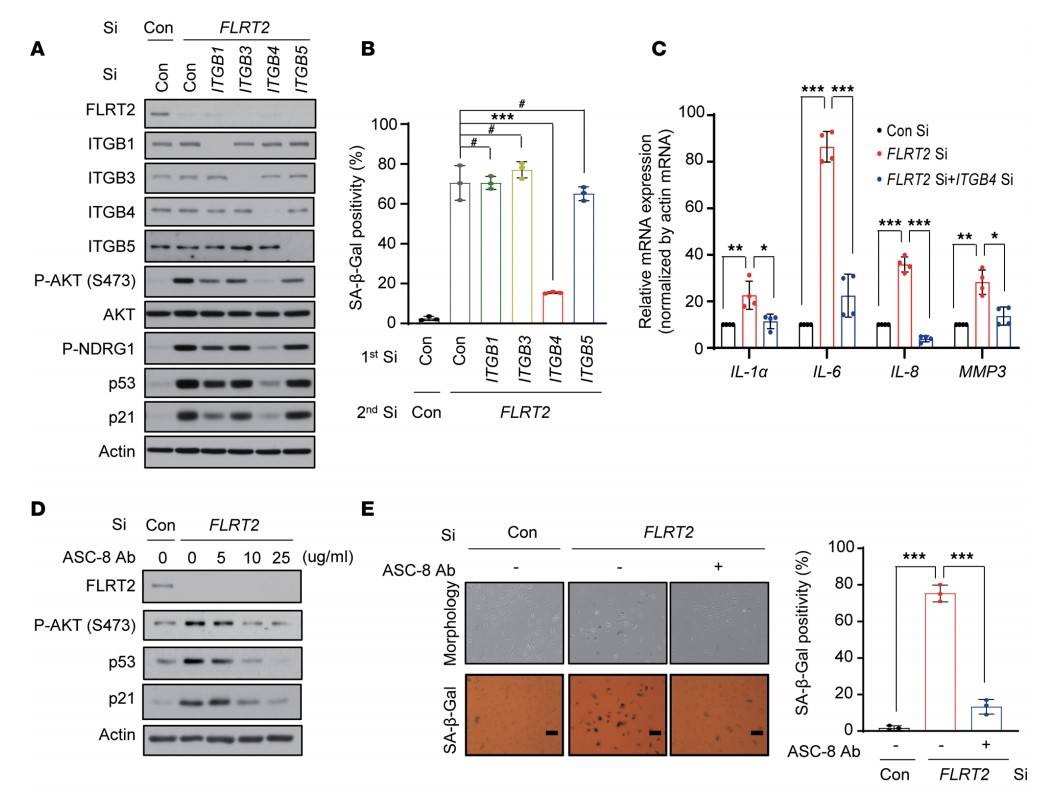
References
- Allard, D., Figg, N., Bennett, M. R., & Littlewood, T. D. (2008). Akt regulates the survival of vascular smooth muscle cells via inhibition of foxo3a and gsk3. Journal of Biological Chemistry, 283(28), 19739–19747.
- Basatemur, G. L., Jørgensen, H. F., Clarke, M. C. H., Bennett, M. R., & Mallat, Z. (2019). Vascular smooth muscle cells in atherosclerosis. Nature Reviews Cardiology, 16(12), 727–744.
- Hwang, H. J., Kang, D., Kim, J.-R., Choi, J. H., Ryu, J.-K., Herman, A. B., Ko, Y.-G., Park, H. J., Gorospe, M., & Lee, J.-S. (n.d.). FLRT2 prevents endothelial cell senescence and vascular aging by regulating the ITGB4/mTORC2/p53 signaling pathway. JCI Insight, 9(7), e172678.
- Spinetti, G., Kraenkel, N., Emanueli, C., & Madeddu, P. (2008). Diabetes and vessel wall remodelling: From mechanistic insights to regenerative therapies. Cardiovascular Research, 78(2), 265–273.
- Wilson, D. P. (2011). Vascular smooth muscle structure and function. In R. Fitridge & M. Thompson Edition, Mechanisms of Vascular Disease: A Reference Book for Vascular Specialists. University of Adelaide Press.